5.3 Thermodynamics
Thermodynamics is the study of energy and its transformations. The First and Second Laws of Thermodynamics form the basis for this science. They can be stated simply as follows: First Law–energy is conserved; Second Law–all available energy cannot be converted to useful work. The First Law is intuitively easy to grasp, as biologists are aware of many kinds of conservation processes. The Second Law, on the other hand, is more difficult to apply but is fundamental to all organisms. Using the example of a living tree, we know from the Second Law that all the energy made available from photosynthesis cannot be converted into useful chemicals for maintenance and growth. There is a certain price paid for the use of energy represented by the structure of the tree. When the tree dies evidence of the Second Law is more obvious. The energy from the sun needed to maintain the tree in an ordered living state can no longer be utilized and the molecules of the tree become scattered in the environment. Schrodinger (1945) has called living things machines that pump out disorder. Further discussion of the Second Law will be found in other modules.
The usefulness of thermodynamics is in the wide range of applications of its concepts and in our ability to represent these ideas mathematically. Thermodynamics was developed to answer practical problems in engineering sciences. The traditional subject areas that are associated with thermodynamic theory are power generation and heating and ventilating (mechanical engineering), industrial chemical processes (chemical engineering), rates of reaction (chemistry), and statistical mechanics (physics). The universality of physical laws makes the use of thermodynamic processes in ecological systems a natural step. Scientists such as meteorologists, physiologists, climatologists, foresters, and agronomists use thermodynamics to study natural and/or biological systems.
5.3.1 Scope of Thermodynamics
Thermodynamics is applied at the microscopic scale to understand the properties of individual particles. Kinetic theory or statistical mechanics, as this subject is known, describes the position and velocity of all the molecules in a system. Our interest here is the macroscopic scale. Although macroscopic theory can be derived from statistical mechanics, historically it was developed first from experimental results. Macroscopic theory describes properties of the system that are general across materials. Furthermore, the variables needed to describe the macroscopic view of the system are few, perceptually obvious (for the First Law), and usually measurable. For example, we may wish to calculate the heat loss of a deer. The surface temperature of the different appendages of the deer would refine our estimates, but the temperature distribution of the cells of the deer’s ear is not necessary to understand the heat energy balance of the animal with its environment.
Included within the scope of thermodynamics are heat energy and mass flow, both of which are important in biology. Energy flow usually involves heat transfer such as a lizard using the sun to elevate its body temperature. The release of water vapor is an important example of mass flow. In order to photosynthesize a green plant must release water vapor to absorb carbon dioxide. This process is called transpiration. Transpiration also affects the heat balance so that the heat and mass flow are coupled processes. Another example of the importance of mass flow is the study of carbon dioxide cycle in the process of photosynthesis. For animals, mass flow in the form of water vapor is commonly used to reduce heat stress. Mechanisms include heat lost during evaporation of water by respiration, sweating, and/or directly through the skin.
5.3.2 Systems Concepts
The focal object is called the system in thermodynamics. The boundaries of the system are arbitrary and need not represent real physical boundaries. The system might be a spider or ground squirrel, but it could be a group of huddling penguins. A plant, a leaf, and a canopy are other examples of systems. An ecologist might very well be interested in an inanimate part of the environment because of the organisms found in that system. Examples are a dead tree trunk, a pond, or the soil. Anything outside of the system that affects its behavior (thermodynamically) is called the surroundings. Identifying the system and its surroundings is usually straightforward. The terms are useful for describing a problem in general terms.
A system is said to be in thermodynamic equilibrium if it is the same temperature as its surroundings. A system is said to be in steady state if its temperature is not changing with time. It is important to consider the time scale here. For instance, the soil temperature one meter down varies only about 1% on a daily time scale but from season to season the variation may be substantially greater. In contrast, consider a leaf that has just become shaded because a cloud covered the sun. The leaf will not receive the radiation energy from direct sunlight and its temperature will quickly fall to a new steady state and thus a new temperature. The same kind of thermal behavior applies to other small objects that do not produce significant metabolic heat such as many insects.
Large objects, for example, a pond, a tree trunk, or a camel may often be in non-steady (transient) state. This is a result of two physical ideas. First, if the system is large it takes a long time for it to come to equilibrium. The temperature of a tree trunk, if suddenly shaded as in the leaf example, would not come to equilibrium for a much longer time. The temperature of the system will be changing but it is changing so slowly that it appears to be in steady state. Even a homeotherm such as a white-tailed deer may have either a net gain or loss of energy at any particular instant during a twenty-four-hour period but its body temperature will only show a variation of about 2∘C. This non-steady state behavior is further complicated by the fact that radiation, air temperature, wind, and humidity (the effects of the environment or surroundings of the system) are also changing with time. Therefore, a system with a large mass may never reach equilibrium. Fortunately, it is possible to describe such systems accurately as we will see in a later module on heat transfer processes.
All real systems and especially biological systems are open. That is, they exchange mass and energy with their surroundings. All organisms not only exchange heat energy with their environment but they must ingest food or absorb nutrients to sustain life processes. A closed system is one in which no mass is exchanged with the surroundings. In biology, environmental chambers are often constructed to approximate closed systems and are used by physiologists to study the metabolism and the water balance of organisms. These kinds of experiments are usually on a limited time scale and will not violate closed system assumptions. An open system may have temperature discontinuities at different points in the system whereas a closed system which by definition does not permit mass exchange will have a continuous temperature distribution. Finally, an isolated system exchanges neither mass nor energy across its boundary. In the special case where heat energy is not exchanged, we say an isolated system has an adiabatic wall which acts as a perfect insulator. Isolated systems have been used to study heterogeneities in the system variables of coupled chemical reactions. Here, people usually are interested in the internal processes of the system.
A reversible process is an idealized thermodynamic process in which no energy is lost. Although no such process exists, the distinction will prove to be useful just as the distinction among open, closed, and isolated systems is useful. In any real energy exchange there is heat loss due to friction that is not recoverable. This fact is one manifestation of the Second Law.
5.3.3 Temperature
Considering the definition of temperature is instructive when applying thermodynamics to biology. Temperature is a macroscopic measure of the molecular vibrations within a system (see discussion of internal energy below). If two systems A and B are in thermal equilibrium with a third body (a thermometer) then A and B are in thermal equilibrium. Formally this is the Zeroth Law of Thermodynamics and can be stated: “there exists a scalar quantity temperature, which is a property of all thermodynamic systems (in equilibrium states), such that temperature equality is a necessary and sufficient condition for thermal equilibrium.”
The three most common temperature scales are Fahrenheit (∘F), Celsius (∘C), and Kelvin (K). These are based on a single fixed point, the unique triple point of water which by international agreement was set at 273.16K. In Figure 5.1, pressure P is plotted against temperature T for some
substance such as water. Solid, liquid, and vapor regions occur at different combinations of temperature and pressure; however, there is a single point at which the three phases occur simultaneously. This fact allows the definition of temperature scales with reference to a unique physical value.
Table 5.1 summarized some of the common temperatures expressed in the different scales. Formulae are given to convert a measurement in one scale to that of another.
∘F | ∘C | K | |
---|---|---|---|
Absolute zero | -459.67 | -273.15 | 0.00 |
Ice point of water | 32.00 | 0.00 | 273.15 |
Triple point of water | 32.018 | 0.01 | 273.16 |
Boiling point of water | 212.00 | 100.00 | 373.15 |
∘F=1.8(∘C)+32.00 K=∘C+273.15
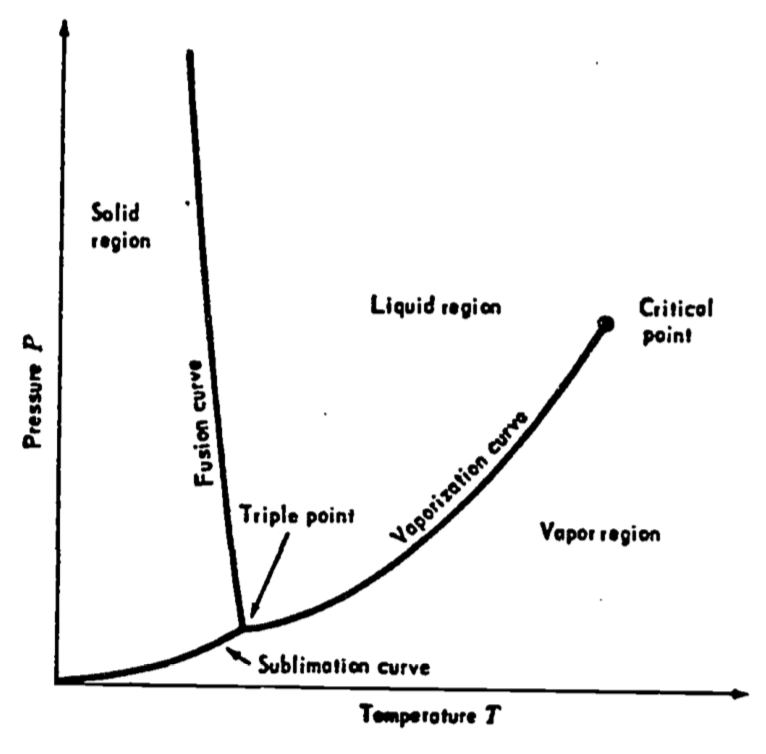
Figure 5.1: A pressure-temperature diagram for a substance such as water. The triple point is the point of intersection of the sublimation, fusion and vaporization curves. From Zemansky, M.W. and H.C. Van Ness. 1966. P. 200.
Now that it is clear how temperature-sensing devices can be scaled, we can briefly describe some of these instruments. Constant-volume gas (hydrogen or helium) thermometers have long been used as the standard thermometric instruments. Liquid in a glass capillary is the most familiar instrument for measuring temperature. Today many temperature measurements are made with electric resistors, thermocouples, and thermistors. For a thermocouple, thermal emf (electromotive force or voltage difference) is measured between a reference junction and a test junction (thermocouple) made of two metals. Electric resistors (platinum for instance) and thermistors (semiconductors) use the principle of the change in electrical resistance of the sensor to determine temperature.
The definitions included above should serve as a basis for thermodynamic language. We can now begin our discussion of a fundamental postulate, the First Law of Thermodynamics.