4.2 The Atomic Theory
Perhaps the most important hypothesis in all of biology is that “there is nothing that living things do that cannot be understood from the point of view that they are made of atoms acting according to the laws of physics” (Feynman 1963). The atoms of which all things are made are moving about in a state of perpetual motion (even at a temperature of absolute zero), exerting forces on each other (attractive when far apart, repulsive when very close), joining into or dissolving partnerships with each other (i.e., forming molecules) when conditions are right and ultimately organizing into such relatively simple collections of molecules as a grain of sand or a lump of coal, or into very complicated collections of molecules capable of writing “Hamlet” or of composing the “Jupiter” Symphony.
It is the job of physics (or let us say, of the biophysicist) to figure out how atoms interact with one another, why certain atoms have the preferences they do for certain other atoms (and the preferences in combining with them in certain particular angles), and what eventually makes these preferences important to sustaining life in its almost infinite variety of forms. To begin this job, we will have to know something about the forces acting on and between these atoms. We will also study the closely allied concept of the energy associated with these forces, which turns out to be a much more powerful method for understanding atomic (or life) processes than if we were to study only the forces.
Before discussing the fundamental forces and associated energies in detail, it will be useful to demonstrate the power of the atomic hypothesis by examining the structure of the water molecule (Fig. 4.1), and the implications of this structure for biology.
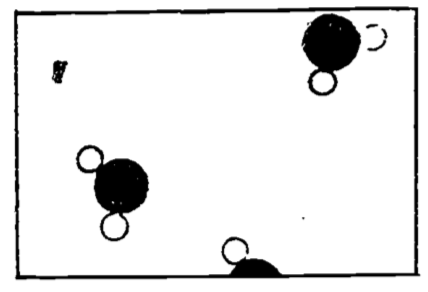
Figure 4.1: Water vapor (steam) (from Feynman 1963).
If we imagine that Fig. 4.1 is an enlarged photograph of a portion of a vessel containing water molecules only, we would infer that the water was in the vapor state, or steam, since we see so few water molecules.
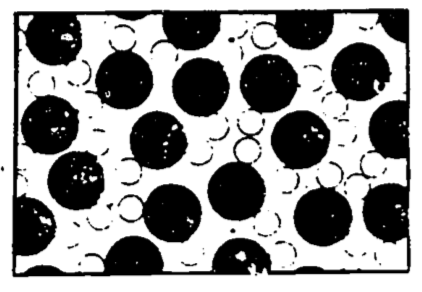
Figure 4.2: Water (liquid) (from Feynman 1963).
Figure 4.2 shows the molecules as they might appear when in the liquid state. Here the molecules are relatively closely packed, but still in a jumble. This state might have been arrived at from the previously illustrated vapor state by several means. We might have just added more steam molecules to force the molecules to be closer to each other (by increasing the density). (We would be required to do this at a higher pressure than existed already in the vessel, else more molecules would leave the vessel than enter it when we were trying to add some molecules.) We might only have decreased the volume of the vessel by forcing a piston into it—this gets the molecules closer together by increasing the density and hence the pressure without adding more molecules. Or we might simply have lowered the temperature of the vessel; this slows down the molecules sufficiently that the attractive forces between them are not overwhelmed by the energy of their motion, and permits groups of molecules to affiliate (or condense out of the vapor) whereupon these groups will respond to the earth’s gravity and join other such groups at the bottom of the vessel in a puddle of water.
If we wait long enough at some appropriate conditions of pressure, temperature, and volume, a so-called equilibrium of the liquid and its vapor will come to pass (if the vessel is isolated). This doesn’t mean everything has stopped. On the contrary, the molecules in both the liquid and vapor states are moving and jiggling violently about. Those molecules in the vapor state experience little force from other molecules, though occasionally they bang into each other or return to the surface of the liquid. Those molecules in the liquid state are held in the liquid by fairly strong attractive forces from their neighbors, but occasionally they gather enough velocity to escape the liquid into the vapor. Equilibrium is said to occur when the average number escaping the liquid per unit time equals the average number returning to the liquid per unit time. If the vessel is open to the atmosphere, those water molecules which have escaped from the liquid will not be likely to return, and eventually all the liquid will evaporate.
The average velocity of the molecules determines the temperature of the substance, the higher the velocity the higher the temperature. When a “hot” object is placed against a “cold” object, some of the faster (or more highly energetic) molecules of the hot object will collide with the less energetic molecules of the cold object, the net effect being that the “hot” molecules slow down and the “cold” molecules speed up. In this event we will say that heat energy has been transferred from the hot to the cold object. Notice from the preceding paragraph, that when the liquid is evaporating, it is the molecules with the higher energies that escape the liquid, leaving relatively lower energy molecules behind. Thus if the vessel is open, so that these high energy molecules are unlikely to return, the liquid will cool. This cooling of the liquid can be sped up by immediately removing newly escaped “hot” molecules in order to prevent their return, such as by blowing them away. “Hence, blow on soup to cool it!” (Feynman 1963).
Figure 4.3 shows a two-dimensional (and hence wrong, except qualitatively) representation of a three-dimensional crystal of ice. An important feature of this diagram is that each molecule has its ordered position in a periodically repeating array. Do not be led by this to believe that the molecules are held so tightly in place as not to be moving.
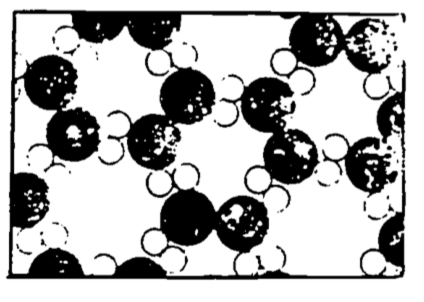
Figure 4.3: Ice (from Feynman 1963).
They surely are not free to slide and move among each other, but they are vibrating in place, so that an occasional molecule can by chance receive sufficient energy from its neighbors to escape the surface of the ice. Notice also the “openness” of this crystal structure, which is partly a consequence of the 105° angles between the hydrogen atoms of the water molecule. When the temperature of the ice is raised to the point where the vibrational energy of the individual molecules breaks the hydrogen bonds (i.e., when the ice melts), the molecules are free to slip into the more closely packed (albeit less structured) liquid arrangement. Water is more dense than ice—hence, ice floats! This is a peculiar property of water (since the solid for most substances is denser than the liquid state) and is of great biological significance.
As the surface water of a lake cools ice forms and floats to the surface. The ice thus formed serves as an insulator against continued, otherwise rapid, freezing of the water below it. In other words, heat transfer from the water to the cold winter environment must take place through an increasingly thicker layer of ice, which greatly slows the freezing of the lake, contrary to what would happen if the ice sank, leaving the surface of the lake constantly exposed to the cold. Thus life in the lake is preserved under the ice layer. Additionally, if ice sank to the bottom, then in spring and summer thawing the heat transfer would have to take place through an increasingly larger insulating layer of water. In fact, many lakes in north temperate climes would likely never thaw, especially after several years of this process permitted the bottom ice layers to build up.
We now have an inkling of the power of the atomic hypothesis. We begin next a discussion of the basic force laws of physics, and will continue to invoke the atomic hypothesis in the ensuing discussions.