9 From pattern to process in the search for generality
Françoise Cardou and Marc Cadotte
How to cite this chapter
Cardou F. and Cadotte M. W. (2023) From pattern to process in the search for generality. In: Cousens R. D. (ed.) Effective ecology: seeking success in a hard science. CRC Press, Taylor & Francis, Milton Park, United Kingdom, pp. 151-172. Doi:10.1201/9781003314332-9
Abstract Ecological systems are amazingly diverse and complex. Scientists that want to make sense of these systems have two choices: to document every blade of grass or mosquito on every continent, or to seek general theories that apply in a range of conditions. With an overwhelming focus on the identification of general patterns, Ecology’s poor track record in finding general theories has become notorious; nowhere has this been so apparent than at the community level. Operating at a scale where contingencies are multiplied and yet too small for general patterns to emerge, the end of the 20th century saw community ecology slated for retirement. This chapter explores how, faced with a crisis, new approaches evolved in this branch of ecology which, by emphasising process over pattern, have achieved at least some generality. We discuss the role of trait-based approaches in these advances, and how their contribution is embedded within a greater discipline-wide theoretical process-first re-organisation. We argue that achieving generality in ecology is at least partially under our control. Though process-first approaches likely have more to give, ecologists should continue to assess progress critically and remain open to changing their approach.
9.1 The pattern-first approach to ecology
Science seeks to understand and predict the natural world. While the wondrous complexity and diversity of biological systems has inspired countless generations of romantics and thinkers, to the scientist, it presents potent challenges. If every biological phenomenon is a unique and special event that is dependent in some way on the particular context in which it is observed (in other words, if it is fully ‘contingent’), then understanding even something so routine as the effect of herbivory on prairie plants could reasonably entail measuring every blade of grass in every prairie on every continent, every day of the year. While this prospect might still attract a few patient and dedicated souls, for many others, this would be a deflating prospect.
General theories are statements about nature that propose specific relationships between observable phenomena and which are thought to apply to a range of conditions (Peters 1980) – for instance, a statement about the effect of large herbivores on graminoid species, independent of climate. Because they have ‘fewer or less restrictive pre-conditions’, general theories contain more information about the world (Peters 1991). Thus, a statement about ‘plant leaf response to physical damage’ contains information about many more individuals than a similar statement about ‘leaf response to physical damage under drought in the rare Western prairie fringed orchid Platanthera praeclara’, which addresses only a small subset of cases. For much of the 20th century, the grinding search for such general theories was thought of as the main mechanism for progress in science. Think of the simple caricature of science (Figure 9.1) where arrows take us from question to hypothesis to experiment to conclusion or theory. By eliminating unsuccessful theories using experiments and observation, successive theories might approach the truth (Niiniluoto 2019).
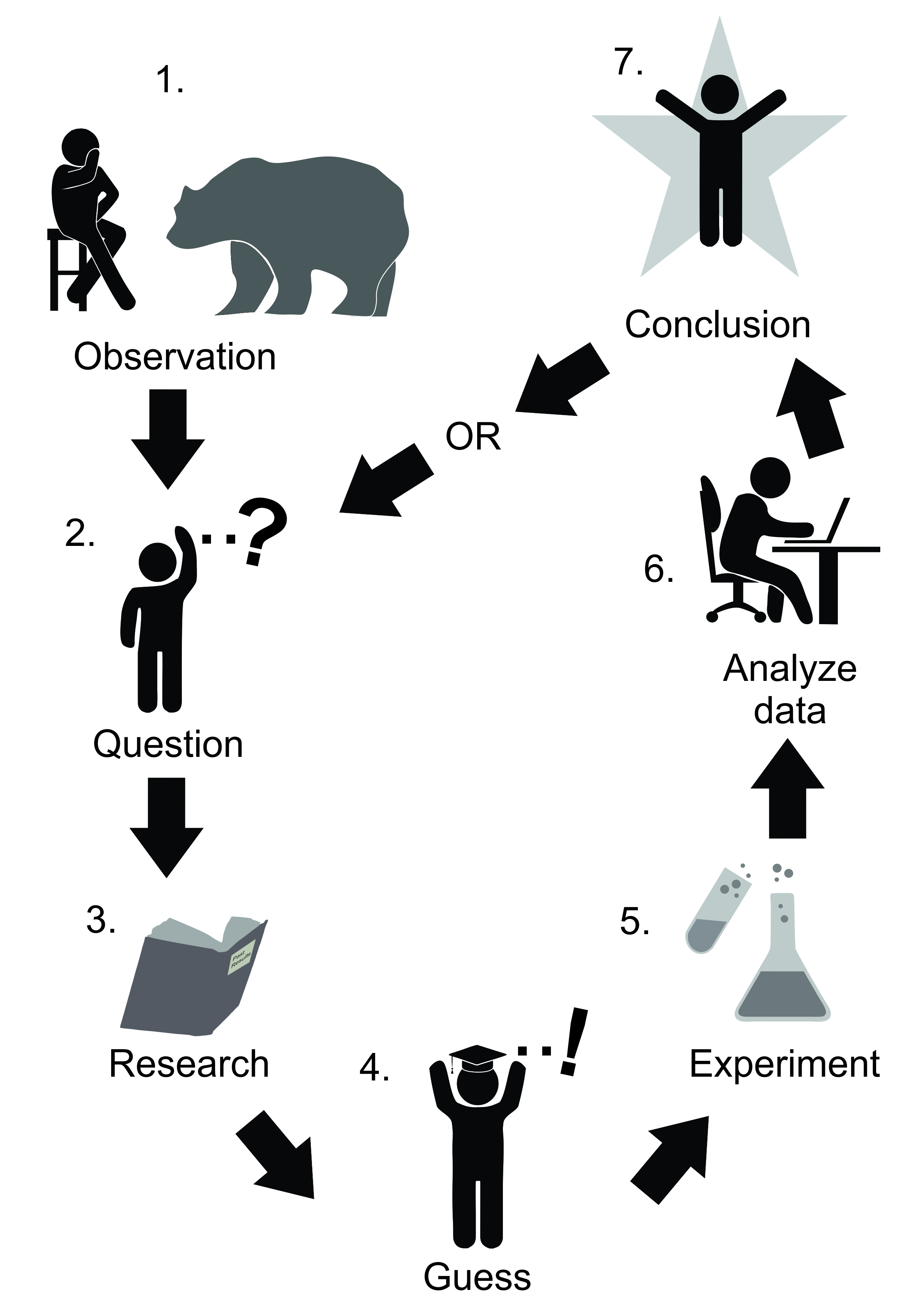
Figure 9.1 According to Popper’s ‘falsificationism’, science progresses by subjecting general theories (statements about how phenomena relate to each other) to testing via experimentation or other means. If the general theory is not supported by testing, it is eliminated from the range of theories that we have about the world. If it is not eliminated, it survives only until it can be tested again.
Where do ecologists typically look for such general theories? In a famous quote from MacArthur (1972) ‘to do science is to search for repeated patterns, not simply to accumulate facts’. Readers of ecological textbooks will be familiar with extensive discussions of canonical ecological patterns like latitudinal diversity gradients, multi-annual population cycles and species-area relationships. The idea is that if patterns repeat predictably in a wide array of ecological contexts, they may be amenable to explanation by single, general all-or-nothing processes. Take for instance the very basic processes of distribution and diffusion of chemicals across membranes with which scientists were able to explain ubiquitous scaling laws in biology (Brown et al. 2004). General patterns are therefore often thought to be good contenders to yield general theories (e.g., Lawton 1999).
For the philosopher Kuhn, scientific progress was anything but assured. He argued that scientific disciplines tended to go through phases, but movement from one to the other was not assured. He called disciplines that do not share an underlying framework or conceptual basis ‘pre-paradigm’ sciences. Here, scientists can work, but this work is neither well-organised nor effective (Godfrey-Smith 2003). If a piece of work emerges that suddenly provides a common model from which further investigation can grow, a field now has a ‘paradigm’, and can establish a new tradition. Kuhn called this phase ‘normal science’, where day-to-day scientific activity might look a lot like Figure 9.1. Although new paradigms can initially yield new and unexpected insight, over time, any new observation that does not conform to the paradigm is taken as a sign of researcher error, rather than a challenge to the paradigm. As more and more evidence accumulates that conforms poorly to the reigning paradigm, scientific disciplines can stagnate, until such time as a crisis arises that opens the door for scientific revolution and the emergence of a new paradigm.
Scientific disciplines can stagnate for a range of reasons, and Ecology has always benefited from a range of critiques seeking to catalyse change (e.g. Peters 1991; Shrader-Frechette 2001). However, at least one has had disproportionately larger impact: in 1999, Lawton published an influential assessment of ecology’s progress towards generality. His view was a pessimistic one. While ecology had yielded some recurring patterns, few of them were generalisable in any useful sense. In particular, he (famously) singled out one subdiscipline as a laggard: for him, ecology’s reputation as ‘soft’ science was largely due to the overwhelming emphasis on community ecology.
Lawton bemoaned the fact that community ecology had few, if any, general patterns. Because here the focus is on the study of ecological phenomena at a scale that is sandwiched between population-level and macroecological processes, he concluded that communities were too contingent, complex and confounded to yield generalities. It should be jettisoned from the ecological program. He believed that population ecology, with just a handful of parameters describing population growth, and macroecology, with few broad environmental and geographical variables controlling range size and diversity, were the appropriate scales of study for ecology to progress. Lawton was not alone in this view. Many scholars felt that community ecology, and perhaps ecology in general, had hit the end of the road and that sustained scientific progress was unlikely, at least unless new ways of thinking emerged (Simberloff 1980; Peters 1991; Ricklefs 2008).
Twenty-five years later, community ecology is still clearly alive and well: it endures as a core and (we would argue) energised subdiscipline in ecology. How did it overcome the crisis illustrated by Lawton’s critique? Although pattern-first approaches have been popular in ecology (MacArthur 1972; Lawton 1999) they are not the only pathway to generality (Cooper 2003; Fox 2019), and are arguably incapable of providing alone the theoretical basis for novel predictions (Cadotte and Tucker 2017; Kraft et al. 2015a).
Community ecology is not the first scientific discipline to tackle complex, confounded and contingent phenomena (Vellend 2016). Rather than be deterred by repeated failures to find general patterns and drawing inspiration from parallel fields like evolutionary biology, community ecology has undergone a rapid shift towards a new source of generality: processes. Beginning with a brief tour of community ecology, we retrace the move from pattern-first to process-first approaches and how it has helped achieve greater generality across three of community ecology’s core goals. Despite these advances, we argue that community ecology is still hindered by the search for single all-or-nothing explanations. We conclude that even in ‘pluralistic’ systems that are contingent, complex and confounded, ecologists have a range of approaches at their disposal to achieve generality. Though process-first approaches likely still have untapped potential to achieve greater generality, ecologists should continue to assess progress critically.
Contingent : Synonymous with ‘context dependent’, referring to situations where relationships vary depending on the conditions – the context – under which they are observed (Catford et al. 2022; Lawton 1999).
Coexistence theory : Posits that species coexistence is regulated by two inequalities: fitness differences (where fitness captures growth and reproduction) and niche differences. According to this theory, two species with similar fitness values need only a small niche difference to coexist, and species with large fitness differences require a much greater niche difference (Chesson 2000).
Ecosystem function : Stocks of energy and materials, fluxes in energy and material processing, and the stability of these fluxes in time (Pacala and Kinzig 2013).
Generality : Property of knowledge (concerning a question, system, species or situation) that is transferable to other questions, systems, species or situations (Fox 2019).
Scientific theories : Predictive and falsifiable statements about nature that propose relationships between observable phenomena (Peters 1980).
Pattern : Regularity in what we observe in nature, or in other words ‘widely observable tendencies’ (Lawton 1999).
Process : ‘A continuous and regular action or succession of actions occurring or performed in a definite manner, and having a particular result or outcome; a sustained operation or series of operations’ (Oxford University Press 2022).
9.2 Community ecology: a brief overview
Any scientific discipline must define a fundamental unit that provides the origin of explanation, or is the thing being explained, whether we are talking about energy, genetic variation or ecological communities. As early as the 19th and early 20th centuries, ecology witnessed the development of contrasted perspectives: proto-ecologists hailing from scientific traditions of taxonomy and systematics had a tendency to classify and categorise plant ‘societies’ into complex hierarchies (Schröter and Kirchner 1896; Braun-Blanquet 1932). In comparison, early ecologists hailing from physiology and natural history tended to focus on functionality (Drude 1896; Warming 1925), a view which, if applied at the individual species or whole community level, led to a famous debate between the so-called Clementsian and Gleasonian views and which we will return to in the next section. Fundamentally, what distinguishes these views is the level of predictability that we can expect from the association that exists among species that form communities.
Communities are now commonly defined as a group of interacting populations in a given area, or the area that contains all interacting individuals (Ricklefs and Relyea 2018; Smith and Smith 2014). Such a definition is of course imprecise and difficult to implement: it requires that we know both the precise extent of each constituent population and have information on the extent to which these populations interact. This is simply implausible. Populations themselves defy a simple delineation of boundaries, and interactions among species can take place across broad spatial and temporal scales. Take for instance the case of migrating birds or predators with home ranges many orders of magnitude larger than that of their prey.
In reality, ecologists do not study communities per se. What they frequently study is an arbitrary area that they believe captures an adequate representation of the types of interactions and mechanisms structuring species abundances and diversity within it, regardless of the trophic complexity of the study question. With this pragmatic approach to the study of communities in hand, we still need to determine what community ecologists typically aim to achieve.
9.2.1 What do community ecologists try to predict and understand?
Ecology as a whole can be defined in a number of ways, and emphasis provided by different authors can lend community ecology a range of flavours. For instance, definitions emphasising the ‘abundance and distribution’ of species used in popular textbooks like Krebs (2000) originate from biogeography. Other definitions emphasise the study of pools and fluxes of energy through both biotic and abiotic components in order to understand the physiology of whole ecosystems (Odum 1953).
Up until relatively recently, a majority of community ecologists would have probably identified most closely with the first of these definitions. From early studies of desert plant assemblages and plankton communities, a large proportion of the work that is still done by community ecologists comes down to understanding a handful of ‘first-order’ community-level attributes (sensu Vellend 2016), such as species diversity and composition, and linking these properties with other site-level characteristics, like environmental gradients (Figure 9.2, I and II).
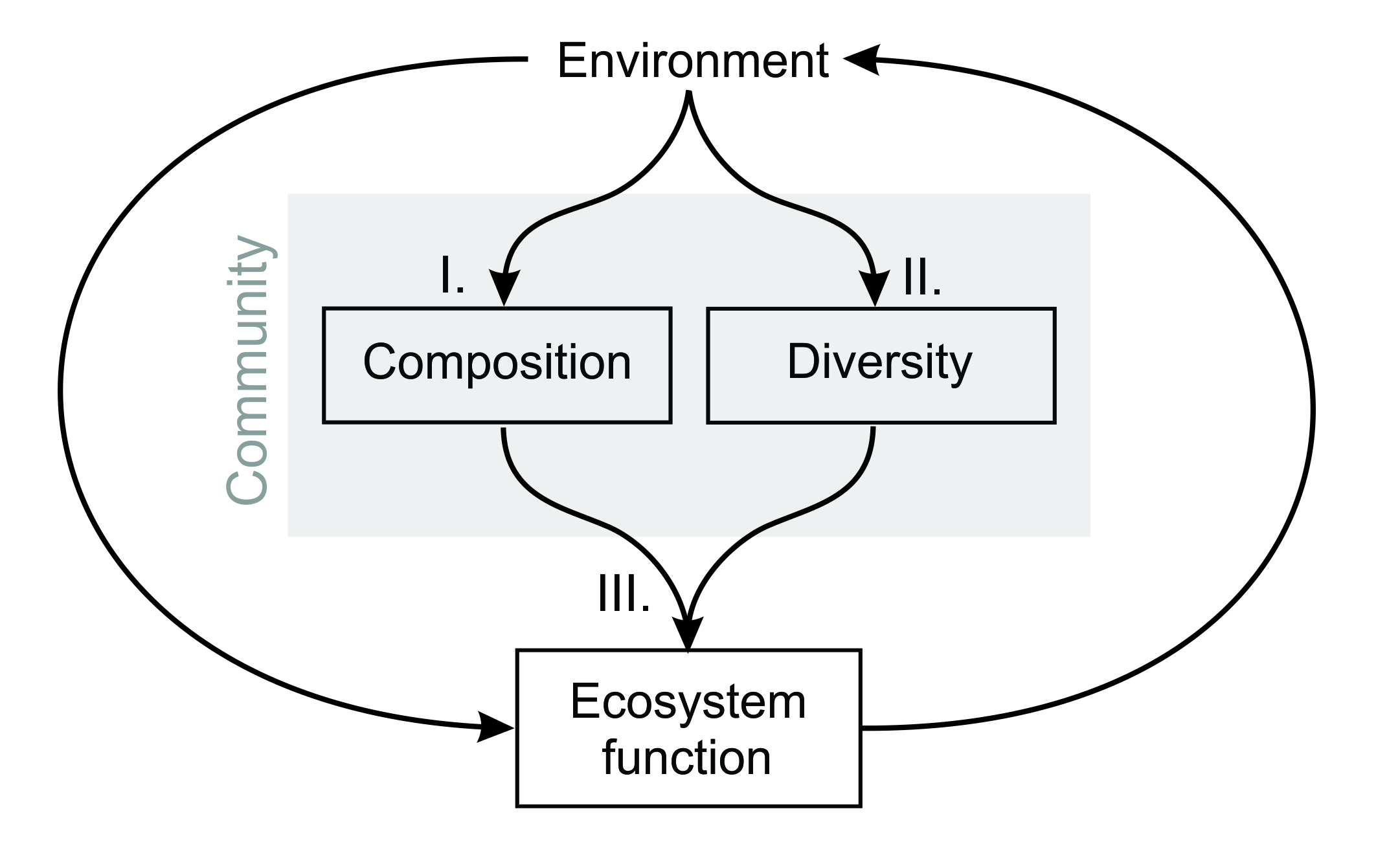
Figure 9.2 An illustration of the main phenomena that community ecologists try to understand and predict. This includes ‘first-order’ properties of ecological communities, like composition (I) and diversity (II), as well as ecosystem-level properties, such as stocks of energy and materials (III).
In contrast, inspired by Odum’s ecosystem-centric view of ecology, another line of enquiry has emerged which is not simply concerned with how species respond to the environment or one another, but how they themselves drive ecosystem processes via close ecological interactions (Figure 9.2, III). Spurred initially by a factious debate on the importance of diversity for the stability of ecosystems, this line of enquiry has bloomed in recent decades into a rich body of knowledge on the multifarious repercussions of community structure on the functioning of ecosystems.
9.2.2 Crisis
Lawton’s critique rightly pointed out that community ecology was an attempt to understand ecological relationships at an awkward scale, sandwiched between population biology and macroecology. As a result, community ecology suffered from the accumulated complexity and contingency of each constituent population, but at a scale too small for this variability to be ignored or abstracted away, or perhaps superseded by larger-scale patterns with regularity. In the 25 years since Lawton’s indictment, macroecology has certainly exploded as an ecological discipline that addresses the geographic, climatic and other large-scale drivers of ecological patterns (Smith et al. 2008). Community ecology, on the other hand, far from fading from the spotlight, seems to have been rejuvenated by not just Lawton’s criticism but other salvos across community ecology’s bow.
9.2.3 The ascent of a process-first approach for ecology
In 2002, Lavorel and Garnier proposed the unification of the approaches that had been developing to understand the abundance and distributions of species (Keddy 1992; Westoby 1998; Grime 1977; Figure 9.2, I and II), with those schemes that attempted to measure the impact of communities on ecosystem processes (Grime 1998; Figure 9.2, III). They did this by moving away from species identities and instead defining species by their suite of ‘response’ and ‘effect’ traits. Here a response trait is a measurable attribute of a species (usually, physiological, morphological or phenological) that influences its response to abiotic and biotic influences, as captured by its growth and reproduction. Effect traits are those that impact ecosystem processes.
With plant ecologists in the vanguard, the unification of community ecology under the common currency provided by traits has had an impact that can hardly be overstated. Because traits provide a mechanistic basis for generalised predictions that are independent of taxonomy (McGill et al. 2006; Shipley 2016), they have opened the door to a generality in our understanding of the selective forces that shape communities that did not exist at the time of Lawton’s critique.
Selective forces that operate on traits are just one of several processes that have been proposed as the explanation for diversity and composition. Against the backdrop of a booming enthusiasm for the deterministic component of community assembly (‘rules of assembly‘) and species coexistence (Chesson 2000; Weiher and Keddy 1995), the last 25 years have also seen the formalisation of ideas surrounding processes that are blind to species’ differences. With Hubbell’s neutral theory of biodiversity (2001) and the advent of metacommunity ecology (Leibold et al. 2004), what has emerged are not universal laws that govern community dynamics in predictable ways, but a finite set of general high-level processes that fully specify the range of mechanisms about which community ecologists make predictions (Vellend 2016).
9.3 The Holy Grail: understanding and predicting community composition
Ecologists commonly chide each other for having what they term ‘physics envy’. This is usually levelled at those who might aspire to a level of generality and predictability that is considered incompatible with the features of biological systems or who are dissatisfied with the current ability to predict variables of interest. Realistic or not, there is a belief among some community ecologists that our ultimate goal is to predict the exact species composition that one might find in a given environment, under given biotic conditions (Lavorel and Garnier 2002; Laughlin et al. 2012; Laughlin 2014). To achieve this would go to the heart of Lawton’s critique and cement the status of community ecology as a predictive science. Not surprisingly, the regularity and predictability of community composition is a topic that has generated both a range of perspectives and fierce debates.
As early as 1909, Spalding was observing that species in desert plant communities seemed to shift when and where they occurred from year to year (Spalding 1909). Around the same time, however, Clements (1916) working in Nebraska was describing the deterministic nature of plant succession and developing the firm belief that there were such things as stable and predictable associations between species. The successional trends that he was witnessing were so regular and predictable that Clements thought plant communities were analogous to organisms, with each constituent species in the community playing a particular and important role, like organs in the body. Much like ambient temperature might affect a person as a whole, Clements thought that external processes acted on the community ‘super-organism’ collectively, leading to predictable associations among species that resulted in climax communities (Figure 9.3a).
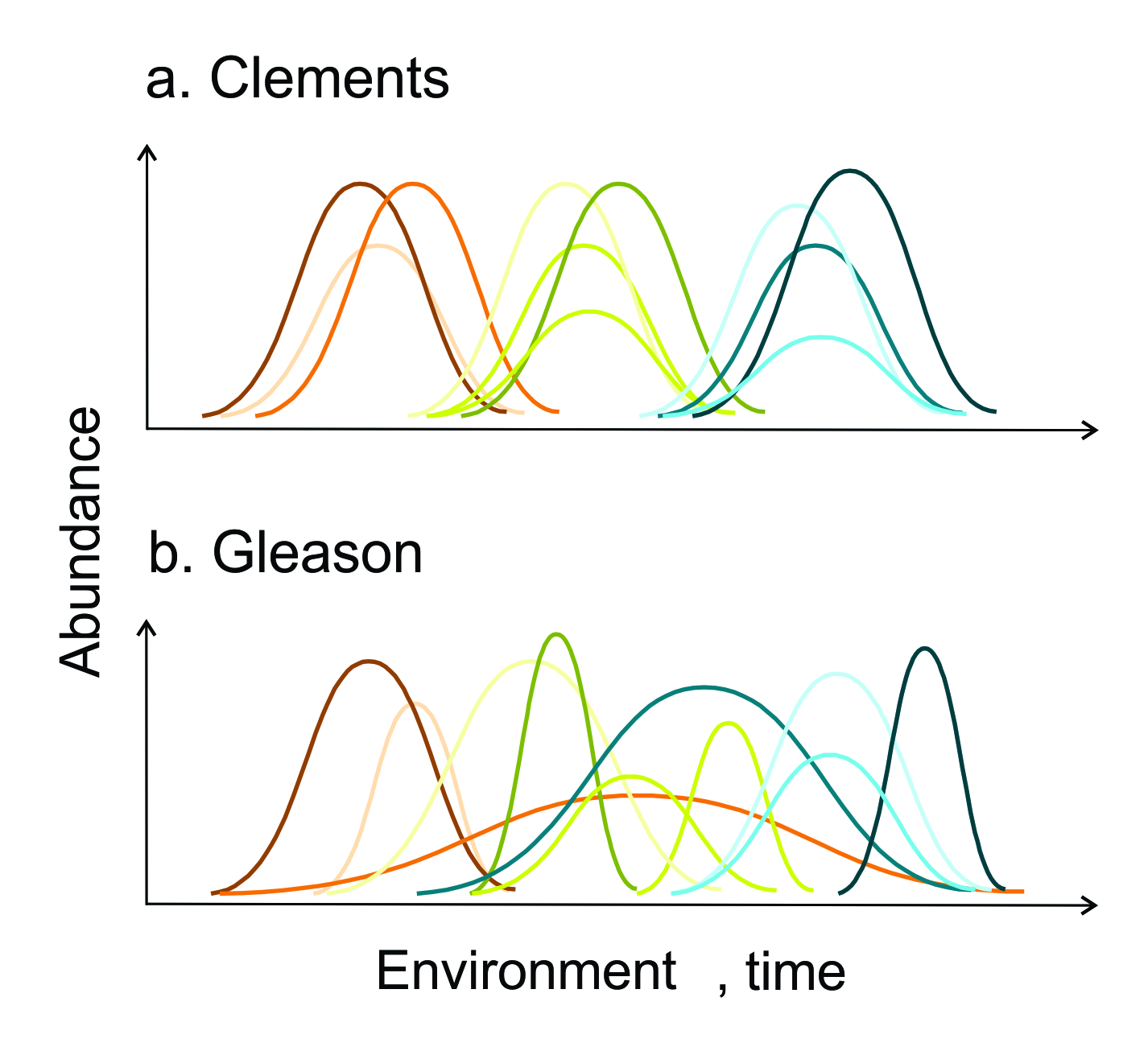
Figure 9.3 The early days of community ecology were concerned in large part with the debate between Clements’ view of communities as stable and predictable ‘societies’ determined by strong inter-species interaction (a). He expected that this should result in stable clusters of species (represented by groups of individual curves in panel a across environmental or temporal gradients). This view was strongly opposed to Gleason’s individualistic view of species’ relation to the environment (b). While the empirical record now chiefly supports Gleason’s view, we also now know that interactions between species can have strong impacts on community composition. Each curve represents a different species.
This view was strongly criticised by Gleason. While Gleason agreed that plant communities exist, can be mapped and studied, he also thought that, consistent with observations like those of Spalding, species respond to the environment individually (Figure 9.3b). Because species could not be expected to co-occur predictably in any general sense, species associations were therefore coincidental, rather than fundamental organic entities (Gleason 1926; Nicolson and McIntosh 2002). By the 1980s Gleason had largely won the debate: his individualistic view of species distributions became a de facto null model for explaining community composition. Any departure from this expectation involving more complex interactions among species had to be demonstrated with solid evidence.
But the success of Gleason’s ideas begs one important question: if species respond individually to environmental gradients, is there any hope of achieving community ecology’s ‘Holy Grail’? Recurrent patterns of compositional change across environments are some of the most striking features of ecological systems. An individualistic view of species’ distribution like Gleason’s could lead to the conclusion that assemblage membership is simply context dependent. Since context dependency is generally a placeholder for a current lack of sufficient explanation, this would be a somewhat unsatisfactory conclusion. On the other hand, if all species respond individually but predictably to the environment, then all that one would theoretically need to predict community composition would be sufficient information concerning the preferences of individual species. The (non)-random structure of communities across environmental gradients has provided motivation and fuel for the search for a general explanation (Whittaker 1960; Laughlin et al. 2012).
9.3.1 Challenge (or strawman) from Hubbell’s neutral theory: do species differences even matter?
Building on Spalding’s initial observations on the transience of desert plant species associations, ecologists have long suspected the importance of stochasticity as a driver of community structure. Yet, the notion that patterns of community composition could be sufficiently explained by processes that are indifferent to the clear differences that exist between species was not formalised until the publication of Hubbell’s ‘neutral theory’ in 2001. Under this theory, species are assumed to have identical niche requirements and to be competitively equivalent: what determines community structure (its diversity and composition) is strictly the outcome of stochastic births and deaths. More specifically, the idea is that a species that sustains some decrease of its population will be statistically more likely to go extinct, regardless of how well it is adapted to its environment. As a result, stochastic deaths (or births) due to, say, the fall of a meteorite can have long-term consequences. Given enough time, neutral theory posits that markedly different community structures can emerge simply by chance.
This was a bold claim and flew in the face of much established wisdom. Ecologists had long been used to devising refined hypotheses to predict species’ responses to their environment that were based on their features. One need only think of the timeless proverb contrasting the oak and the reed and their ability to withstand storms based on their stem properties. What was especially remarkable was that Hubbell’s theory proved able to reproduce species abundance distributions in high-diversity communities quite well (Hubbell 2001). Could community structure really be contingent ‘all the way down’, as Hubbell’s theory suggested?
Neutral theory has had two distinct impacts on ecology research. The first has been to serve as a foil against the overwhelming reliance on deterministic niche-based processes to explain differences among communities. Here, the response was swift and largely negative (e.g. McGill 2003), highlighting, for instance, the fact that species niche differences are easily observable and demonstrable. However, beyond the apparent strawman presented by neutral theory was its second impact - neutrality as an emergent process.
While species have differing adaptations, there are a suite of mechanisms and influences that can equalise fitness such that species with, for example, competitive differences might appear as though their interactions are governed by neutral processes. Any process, either density independent or density dependent, that reduces reproduction or increases mortality can potentially reduce competitive differences, allowing species to persist together (1) if these external influences create the conditions for coexistence (i.e. fitness differences lowered to the point where the positive influence of niche differences can be realised) or (2) if these species exhibit very little niche difference, then this persistence can appear as apparent neutrality (Chesson 2000; MacDougall et al. 2009; Chave 2004).
9.3.2 Predictive community-environment relationships based on processes
If Hubbell’s expectation of species’ equivalence is so unrealistic, then how should we capture species’ differences in a way that can express the deterministic selection forces to which they are responding? A species’ name tells us very little about how it interacts with the world. Rather, it is the measurable physical and biochemical aspects of an individual’s phenotype (traits) that dictate how it perceives and responds to environmental stimuli and how it interacts with competitors, predators, parasites, pathogens and microbial mutualists (McGill et al. 2006; Cadotte et al. 2011). Traits are functional when their variation has demonstrable influences on species performance or other trophic levels or processes like nutrient cycling. By linking traits to species performance across environmental gradients, we can predict the composition and direction of community change because we can evaluate the relationship between, say, the abundance of species that have different wood densities and a soil moisture gradient (Laughlin et al. 2012).
Take, for instance, the change in forest composition that occurs predictably with increasing elevation: this is a regular pattern in temperate environments. In the Smoky Mountains (Tennessee, USA), this trend can be articulated as a transition between Tsuga canadensis at low elevations to Acer rubrum at mid-elevations and Pinus rigida at high elevations (Whittaker 1956). Because this specific group of species does not occur on mountainsides located in Japan or Austria, the previous sentence provides no information as to what forest composition we might find as we climbed these other mountains. In other words, this is a pattern without process and does not provide a general prediction that can be tested in other biomes. However, it does become predictive when we articulate this pattern in terms of the traits that might respond to selective forces at play along this and other altitudinal gradients, resulting in lower water evapotranspiration and greater shedding of snow at higher elevations.
More specifically, such trait-environment correlations capture two interrelated processes. Firstly, the change in trait values reflects the fit between trait values and local environmental conditions. This is the signal of the selective forces that progressively sift out species with ill-adapted traits. Secondly, species fitness, which is influenced by trait values, results in changes in competitive hierarchies, and an inferior competitor could persist in a suboptimal environment in the absence of a species with better-adapted traits (Cadotte and Tucker 2017; Kraft et al. 2015a).
As they have done since the days of Clements and Gleason, community ecologists will no doubt continue to debate the importance of deterministic and stochastic processes in community assembly. The emergence around the same time of both functional community ecology and neutral theory shows that this is not a debate that can be resolved by single decisive tests of each theory. As in population genetics, ‘drift’ within communities is more important in small communities. For their part, trait-based approaches have yielded a number of robust trait-environment relationships that successfully generalise across broad sets of environmental conditions, like water availability, shade or altitude (Poorter et al. 2019; O’Brien et al. 2017; Delhey 2019). What neutral theory really highlighted was that we cannot assume the supremacy of deterministic processes and that, thanks to Chesson’s (2000) expansion of coexistence theory, species interactions fall along a continuum from approximating neutral to very deterministic processes. In other words, both processes co-occur continuously and predictably.
9.4 More than one path: understanding and predicting diversity
The core of community ecology is focused on testing hypotheses about not just the kinds of organisms that we might find in a given place, but their diversity as well. Specifically, for much of the field’s history, community ecologists have developed theory and collected data focused on the number of species in a community.
As early as the 19th century, biogeographers knew that surveys carried out over larger spatial extents would yield a higher number of species (McIntosh 1986). From terrestrial plant communities to freshwater lakes, early quantitative ecologists set about counting how many species might be found if they sampled increasingly large areas (Arrhenius 1921). Formalised into an equation, the resulting relationship yields a characteristic curve (a so-called ‘species-area’ curve; Figure 9.4a) that allowed scientists to make educated guesses about the quantities of species that might be present in areas they had yet to sample. Because such curves tend to share a basic shape, reflecting the balance of common species relative to rare ones, the species-area relationship became known as one of the canonical patterns in community ecology (Preston 1960). Even today, this relationship is still one of community ecology’s very few robust and general patterns (Lawton 1999), holding up across several scales. Its impact on the next several decades of (community) ecology research can hardly be overstated.
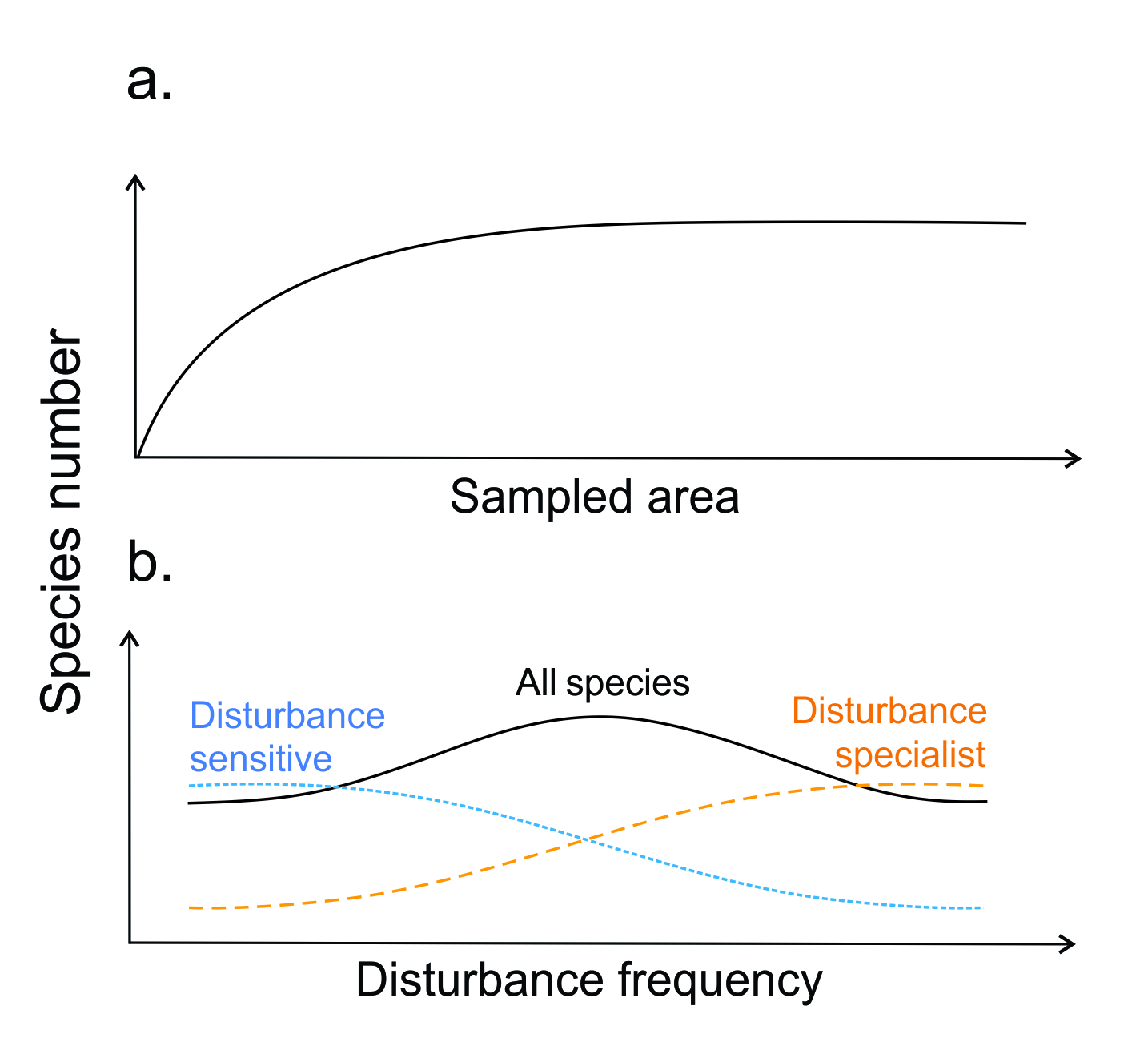
Figure 9.4 Ecologists have tried to find generalisable relationships between diversity and many environmental features. Some have proven robust, like the relationship between species number and sampled area (a), while others like the ‘intermediate disturbance hypothesis’ (b) have been shown to produce divergent patterns based on the traits of species in the species pool.
MacArthur and Wilson were the first to really put the species-area relationship to work. Building on Wilson’s work on ant communities, the pair hypothesised that in an archipelago, the number of species that might be found on a given island would be determined by its total area. Because immigration and extinction are dynamic processes that depend both on the isolation of the island from the mainland (via propagule pressure) and the number of species already present (via competition), the dynamic process of arrivals and extinctions would maintain the number of species in each community around an equilibrium that, despite turnover in species, could be predicted based on just a few parameters (MacArthur and Wilson 1967).
9.4.1 Diversity patterns depend on species pool
Following MacArthur and Wilson’s lead, community ecologists set about exploring many other potential predictors for the number of species that might be found in a given area, beyond its extent and its isolation. In 1918, Thienemann had predicted that communities in areas with a greater diversity of environmental conditions would be composed of a greater number of species. The species richness-heterogeneity relationship has turned out to be another example of a robust and predictive relationship in community ecology, holding up to scrutiny across both plant and animal communities (Stein 2014; Allouche et al. 2012). If we were to stop the roundtable here, one might get the impression that the search for predictors of diversity has on the whole provided community ecology with a firm basis from which to derive general theories. As it turns out, Lawton had ground for concern.
The intermediate disturbance hypothesis (IDH), formulated by Connell in 1978, predicts that diversity should peak at disturbance levels that are moderate enough for sensitive species to survive, but frequent enough to allow disturbance specialists to still colonise, yielding a diversity peak at intermediate disturbance intensities (Figure 9.4b). That this prediction tracks well with the experience of many ecologists, particularly those working in temperate plant communities, is probably not independent of the speed at which this prediction has become common ecological wisdom, or of its tenacity in entry-level ecological textbooks (e.g. Krebs 2000). But while this is probably one of the most frequently tested hypotheses in ecology, there is good reason to doubt its generality. Meta-analyses of empirical results have shown that diversity-disturbance relationships are in fact not peaked or general (Mackey and Currie 2001).
There are conceptual reasons why we might expect diversity-disturbance relationships to have different shapes in different ecological situations (Cadotte 2007). In its original formulation, the IDH assumed that species would be drawn from a pool where species are evenly distributed between disturbance-sensitive and disturbance-specialist species. In this scenario, a progressive increase in disturbance might thus very well lead to a progressive shift from one group to the next, as predicted. There is however no a priori reason why a regional pool would contain even proportions of either group: after all, adaptation to recurrent disturbances depends on suites of traits that can evolve, and thus it is entirely reasonable to expect greater proportions of disturbance specialists in disturbance-prone environments. In this case, rather than a peaked relationship, one would therefore expect a directional increase in diversity with increasing disturbance intensity (Cadotte 2007).
It would be a mistake to think that the particular failures of the IDH are its alone: other hypotheses that attempt to predict robust trends in species diversity along complex ecological gradients have also yielded mixed or weak evidence. For instance, diversity was also long thought to peak at intermediate levels of productivity, but this effect has failed to generalise robustly outside the plant communities where it was first addressed (Mittelbach et al. 2001). Underlying these weak relationships is the fact that they are based on hypotheses that make assumptions about the composition of the species pool: in other words, they rely on a selection mechanism that will yield different results depending on species identity, and as such, their predictions are contingent on the properties of those species that are available to colonise (Ricklefs 2008).
9.4.2 Matching diversity measures to hypotheses to capture processes
If we looked closely at many of the earliest community ecology theories, we would see that they are ultimately based predictions about the effect of numbers and relative abundance of species on species’ niches and niche differences (Chesson 2000). For example, Lotka-Volterra competition models included a parameter describing the strength of the magnitude of the reduction in population growth of one species on another (Lotka 1925; Volterra 1931), assuming that species are likely to coexist if these impacts are low relative to intraspecific density dependence (Chesson 2000); this is what we expect if species occupy distinct niches. Similarly, one of MacArthur’s fundamental insights was that species co-occur and coexist only when they use different resources by partitioning niches, also commonly referred to as Gause’s principle (MacArthur 1958; Gause 1934).
Despite this, we still routinely use species richness as a surrogate for niche differences (Box 9.2). In essence, we assume that a greater number of species should result in more niches being represented, or in a greater amount of niche space. This poor match between the mechanisms underpinning our hypotheses and what we measure - the patterns we seek to explain - have existed in ecology for much of its history. The problem with such surrogacy is that studies which failed to support the intermediate disturbance hypothesis (for example) did not disprove the underlying mechanisms, but rather were unknowingly testing for the relative numbers of species that occupy different niches (e.g. disturbance-sensitive versus disturbance-specialist species).
There are many ways to quantify the diversity of species in a given community. Species richness provides a first-cut measure that simply tallies the number of species present in a community. In cases where we only know which species were present or absent at a site, this is the best we can do. But species’ relative abundances are also an important feature of community diversity, and can vary markedly even when species richness remains constant, as illustrated in Figure 9.5.
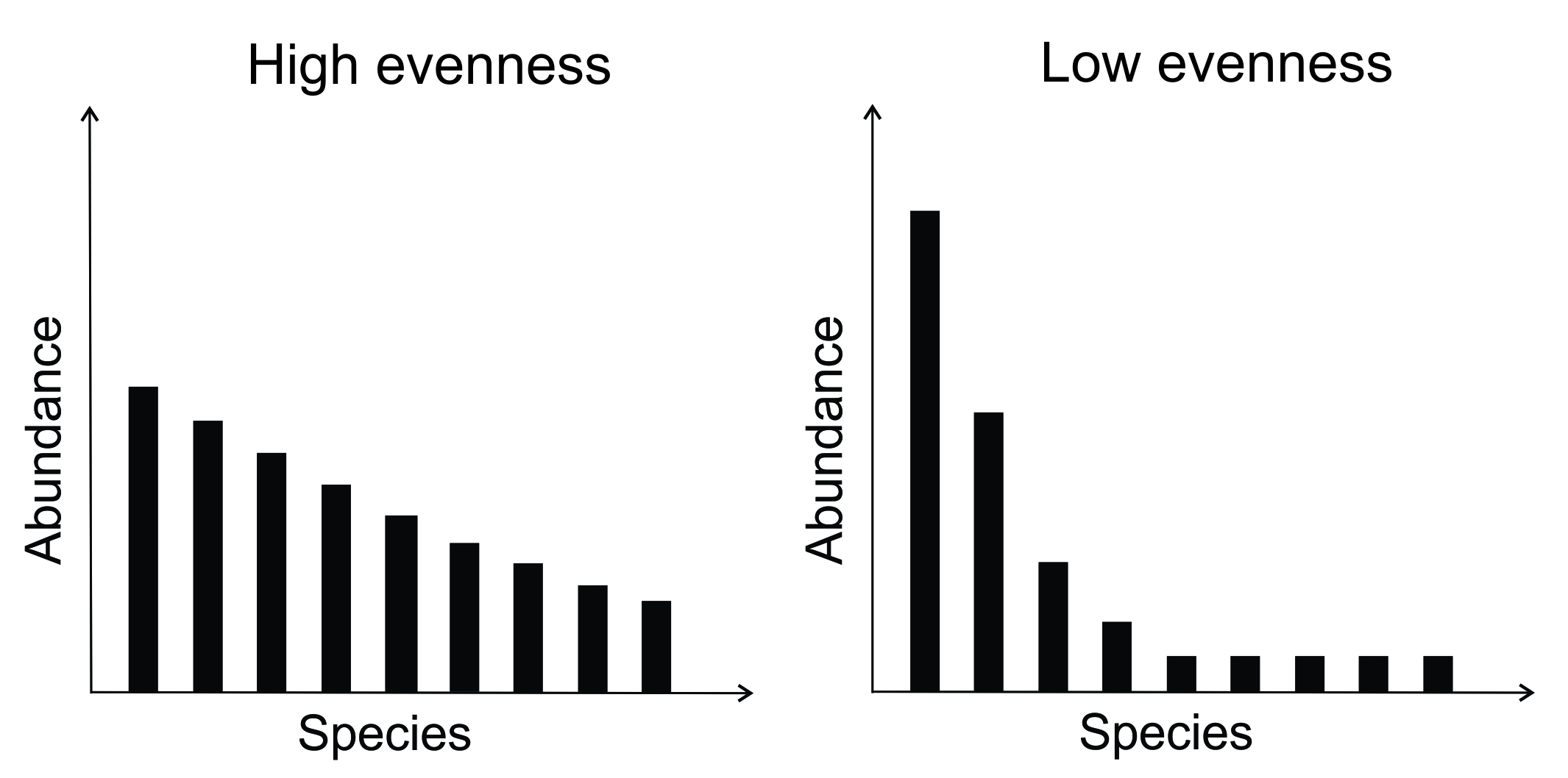
Figure 9.5. Illustration of the principle that either high or low evenness can be found in communities with similar species richness.
A number of indices exist that capture species’ abundance-weighted diversity (evenness). Popular examples include the Shannon-Weiner index and Simpson’s index. The merits of these different indices were a recurrent topic of debate among ecologists through the 1980s and 1990s. We now generally agree that species richness and measures of evenness capture different facets of species’ abundance distribution in a community: they are complementary tools to common questions, and new unified measures based on Hill numbers now allow the computation of diversity measures that incorporate evenness in a variety of ways across a common mathematical formulation (Chao et al. 2014).
Importantly, the critique levelled at indices like species richness in the main text hold equally for all other measures of diversity. When they are based on taxonomic species identity, these indices offer equally poor ways of testing hypotheses that are implicitly based on species’ niche differences. Since the advent of functional community ecology, a full suite of both functional and phylogenetic (based on shared evolutionary ancestry) alternatives to these measures have been developed, such that it is now possible to describe trait distributions to the same level of detail. Incorporating functional and phylogenetic information into diversity measures provides a basis to account for species dissimilarities while still calculating richness or evenness type measures (Mason et al. 2005; Chao et al. 2014; Cadotte et al. 2010).
This type of problem was fully exposed by a seminal paper by Webb showing how the phylogenetic distances among species could be used to assess whether communities were comprised of more closely or distantly related species than expected by random draws from a regional species pool (Webb 2000). Because we do not always know which traits are important for a given species and because functional characteristics tend to be retained within phylogenies, phylogenetic differences provide a holistic proxy for niche differences: these distances can be assessed at the community level in a way that is independent of differences in the number of species. For instance, phylogenetic dissimilarity among species were hypothesised to reflect changes in the relative importance of competition (and thus niche differences) versus environmental filtering (e.g. Bässler et al. 2016).
As we saw in the previous section, predictable relationships between traits and environment allow us to make educated guesses about the presence or abundance of individual species, based on their traits, and about the average trait values of entire assemblages (Laughlin et al. 2012; Laughlin 2014). Species traits and phylogenetic distances are complementary approaches (see Box 9.2; Cadotte et al. 2019) that both connect more directly to the processes underlying coexistence than species richness. For instance, returning to our example of how disturbance shapes communities, a number of studies have shown how variation in disturbance alters both the phylogenetic and functional trait composition of communities in predictable ways (e.g. Helmus et al. 2010). These new approaches to measuring community diversity have opened the floodgates for empirical tests of community assembly mechanisms.
9.4.3 Inferring process from (diversity) pattern: a many-to-one problem
The abiotic environment can influence species fitness directly, by limiting growth and reproduction through constraints that are common to all species in a community. This is termed ‘environmental filtering’. The abiotic environment is also ultimately the source of resources that support populations. For plants, for example, local pools of nutrients like nitrogen and phosphorus, along with water and light fuel growth. As these and other resources are consumed or occupied, they begin to limit population growth: this is the stage at which we expect there to be a shift in the processes driving community assembly, away from environmental filtering and towards competition between individuals within and across species.
Of course, because of local environmental heterogeneity and variability, and because different species are unlikely to be limited by the same resources, no single species has the capacity to consume all available resources. This means that species that can access resources unavailable to others, or are limited by different resources, are able to coexist (Tilman et al. 1982; Gause 1934). As a result, species that are similar in their niches, and by extension their traits, are likely to either face competition resulting in competitive exclusion or will persist neutrally if they have relatively equivalent fitness (Chesson 2000; Adler et al. 2007). Either way, these species will not deterministically coexist. Instead, coexistence will be more likely between those species that exhibit trait differences. Evidence from successional studies generally confirms that as ecosystems become more diverse, interactions play out, heterogeneity increases and communities become more diverse in their traits and more distantly related (Meiners et al. 2015; Li et al. 2015).
It is now routine for ecologists to assess whether communities contain species that are similar or dissimilar to one another (Cadotte et al. 2019). These community-level dissimilarity patterns are typically assessed against randomly generated communities that are sampled from a larger regional species pool. Communities that are functionally or phylogenetically underdispersed (i.e. containing species that are more similar to one another than expected by random sampling) are often assumed to have adaptations allowing them to persist under a severe environmental regime (i.e. environmental filtering; e.g. Bässler et al. 2016). Conversely, communities that are overdispersed are presumed to reflect the outcome of competition, specifically limiting similarity, that selects for dissimilar species.
The problem with these inferences is that these mechanisms (environmental filtering, limiting similarity) cannot be directly assessed from changes in dissimilarity. Different mechanisms and processes can lead to convergent community phylogenetic and functional diversity patterns (Mayfield et al. 2010; Cadotte and Tucker 2017). For example, species facilitation, small-scale soil heterogeneity and limiting similarity can all result in overdispersed communities. Further, different processes can act on different elements of species’ phenotypes, meaning that some traits might be underdispersed because of convergent evolution while they are dissimilar on other trait axes that promote coexistence (Cadotte et al. 2019).
This is a potent example of community ecology’s many-to-one problem. Because different processes can lead to the same patterns (Lawton 1999; Vellend 2016), additional information or experiments are required to rule out or account for potentially co-occurring mechanisms. Without these, reliance on patterns of dissimilarity can lead to erroneous inferences about process, even when measures of dissimilarity are well aligned with niche differences. For instance, to differentiate between different community assembly mechanisms, additional information might be needed on environmental heterogeneity, how individual species abundances covary with environmental gradients and the presence/absence of other species (Cadotte and Tucker 2017). One might even use experiments to determine how species respond to environmental gradients in the absence of competition (Kraft et al. 2015b). Even as community ecology has pivoted towards a process-first approach, the search for all-or-nothing explanations continues to be a stumbling block.
9.5 A little bit of everything all of the time: ecosystem function
The term ‘ecosystem function’ classically refers to three types of ecosystem properties: stocks of energy and materials, fluxes in energy and material processing, and the stability of these fluxes in time (Pacala and Kinzig 2013). In contrast with the challenges encountered in preceding sections, it is noteworthy (and perhaps, to be expected) that attempts to understand how communities affect ecosystem functioning have been process-driven from the start. Still the reliance on species richness in experiments has nonetheless created a mismatch between the measure of diversity employed and the hypothesised mechanisms. In addition, this area of research continues to be hindered by a search for all-or-nothing mechanisms, and by a narrow focus on a subset of ecosystems functions.
From an ecosystem view, plants represent a single functional group that converts energy, light and nutrients into organic matter. This means, first, that plants determine the amount of energy available to all other players in the ecosystem, and second, that their contribution to ecosystem functioning can be assumed to depend on a common set of characteristics. As a result, the study of ecosystem function through the lens of community ecology has been overwhelmingly focused on terrestrial and plant communities. Of course, even in the 1950s, ecologists (not to mention farmers and foresters) knew very well that plants could determine ecosystem properties like soil fertility: farmers have for centuries increased yield by alternating between crops and leaving fields fallow. However, ‘how’ and ‘how much’ plants affect ecosystem functions are questions that have generated at least two fruitful but (until relatively recently) largely orthogonal perspectives, with each side favouring one causal pathway over the other.
9.5.1 The perspective from mathematical ecology
In the 1970s and 1980s, some community ecologists became embroiled in a series of raucous debates surrounding a set of related questions. Several authors had previously converged on the hypothesis that more diverse communities should also exhibit more stable ecosystem properties. When research turned up mixed evidence and weak patterns, the debate eventually shifted to a narrower question. Setting aside their stability in time, could diversity predict ecosystem properties?
Favouring experimental approaches, ecologists of the 1990s set about assembling grassland communities to see whether those with higher numbers of species would turn out to be more productive (Naeem et al. 1994; Tilman et al. 1996; Hector et al. 1999). In general, they did! Of course, these experiments, carried out in tightly controlled environmental conditions, creating relatively homogeneous conditions that likely limited niche opportunities and once again focused on species richness as a proxy for underlying niche differences. They assumed that richer communities would also contain species with more different niches, allowing them to make more efficient use of resources (‘niche complementarity’) and increasing the likelihood that they might establish mutually beneficial interactions.
With such a tenuous link with species’ actual contribution to ecosystem function and little means of distinguishing the effect of diversity per se from the disproportionate contribution of particular species, this line of research soon came under heavy criticism from researchers who had already been chipping away at the problem in natural and agricultural systems (Wardle 1999; Huston 1997; Grime 1997). After all, in natural conditions, the most productive ecosystems are often glaringly species poor. Though community ecologists quickly devised methods to isolate the effects of biodiversity per se (Loreau and Hector 2001) and to capture directly species’ niche differences (e.g. Cadotte et al. 2013; MacIvor et al. 2018), opposing evidence from experiments and in situ studies reinforced disagreements.
9.5.2 The perspective from ecosystem ecology
Meanwhile, ecosystem ecologists and community ecologists had been peeling back layers of abiotic and biotic drivers to reveal complex causal hierarchies that explain local ecosystem properties like productivity and decomposition in natural systems. They were able to show not simply that species composition matters for ecosystem function, but that this impact can be explained by species fundamental properties (effect traits) like growth form and level of investment in various plant structures (Sims and Singh 1978; Lavelle et al. 1993). Because these properties are also involved in how species respond to environmental conditions, they generate biophysical feedbacks that can stabilise ecosystem properties or shift them into entirely new states, with ecological succession being just one of many examples (Vitousek and Reiners 1975; Hobbie 1992).
Biomass is not usually evenly distributed among species within communities. Thus, there was reason to suppose that species would affect ecosystem properties to different degrees, and proportionally to their contribution to the total biomass of the community. This insight was formalised by Grime (1998) under the name ‘mass ratio hypothesis’, and proved critical for the integration of effect traits into mainstream community ecology. In an influential study of a grassland chronosequence, Garnier et al. (2004) demonstrated that, weighted by the contribution of each species to total community biomass, species’ traits could explain (once the effects of environmental heterogeneity are accounted for) upwards of 70%, 80% and even 90% of the variation in ecosystem properties. From this point forward, there was great hope that community ecology might close the loop between the response of community composition to environment change and the repercussions of these changes for ecosystem functioning (Figure 9.2).
9.5.3 All-or-nothing explanations
Global environmental change is associated with pervasive shifts in environmental conditions, accelerating land use change and the increased movement of species from place to place. Even while global losses in biodiversity are incontrovertible, there is also clear evidence that species diversity is just as likely to increase as to decrease at local scales where the close species interactions that matter most for ecosystem function occur (Vellend et al. 2013; Dornelas et al. 2014). But while diversity might appear stable at small scales, communities are undergoing substantial changes in composition (Blowes et al. 2019). Anticipating the effect of global change on ecosystem services, and therefore, on ecosystem functioning, has become one of the great challenges of community ecology.
Given the extensive work done by scientists in experimental settings and in the field, we might have been forgiven for thinking that this area of research would be more than ready to step up to the plate. However, protracted debates between proponents of community composition versus diversity as the main driver of ecosystem function continue to span theoretical, methodological and even sometimes ethical grounds. Though both sides have agreed that plants affect ecosystem processes through their traits (Hooper et al. 2005), ecologists still routinely focus and emphasise the predominance of single all-or-nothing drivers of ecosystem function (e.g. Hooper et al. 2012). Moving forward requires integrating these various traditions to understand which of the three causal pathways in Figure 9.2 predominates, at which scale and in which situations. This may not be entirely enough, either.
On both sides of this debate, ecologists have overwhelmingly focused on one single ecosystem function: productivity. Though studies on occasion have extended their focus to other functions in which plants are known to be involved, like decomposition and soil respiration (Spehn et al. 2005; Cardou et al. 2020), this remains a woefully small subsample of all ecosystem functions on which we depend for ecosystem services. Global environmental change is driving rapid ‘rewiring’ of biotic interactions (Bartley et al. 2019), and it is not clear that community ecology (as we have described it thus far) is ready to tackle this challenge.
9.5.4 Pending challenge: when the pattern is the process
From predator-prey systems to complex food webs, communities that span more than one trophic level have always been recognised as falling under the umbrella of community ecology. Yet, despite the fact that vertical plankton communities provided some of the earliest examples of community ecology (Forbes 1887), the study of communities that include trophic interactions (‘vertical’) versus those that only examine patterns within a trophic level (‘horizontal’) have since largely gone their separate ways.
One reason for this split is most likely a methodological one: sampling across trophic levels requires broad and diverse skill sets, including different sampling techniques and complementary taxonomic identification abilities (Seibold et al. 2018). More fundamentally, these two fields also typically test different hypotheses and rely on different definitions of what an ecological niche is. For horizontal community ecologists, the niche refers to the various strategies that organisms can adopt to ensure their own survival under various environmental conditions (Hutchinson 1978) while vertical community ecologists understandably think of it as the role that species play within the system: it is defined by species-to-species interactions, such as predator and prey (Elton 1927). Vertical communities therefore challenge theories developed for horizontal community in fundamental ways.
Within-community interactions in horizontal communities are assumed to be primarily defined by competition: while different pairs of species can be involved in different levels of competition, the interaction is assumed to be symmetrical, with both partners incurring similar cost from their shared overlap. Vertical communities are defined by the asymmetrical exchange of energy between species: interactions can be assumed to be diverse, strong and negative as well as positive. Another assumption of modern coexistence theory is that species success is the additive outcome of direct pairwise interactions, but strong indirect effects are widely described in food webs (e.g. Godoy et al. 2018).
If species coexist and persist not through varying responses to shared constraints on their fitness but instead as a direct result of trophic exchanges, then the structure of these interactions provides another entry point into community assembly. So far, however, the study of ecological networks has focused overwhelmingly on describing network structure and identifying linkages with other network-level measures, like ‘stability’ (Marjakangas et al. 2022). Because the latter case treats interactions (ecosystem processes) as the explanatory variables and stability (coexistence) as the response, the resulting knowledge maps awkwardly onto the insights pursued by horizontal community ecologists, and for whom properties of communities like diversity and composition are the variables among which an explanation of ecosystem process might be found.
Still, several recent studies attempt to bridge the conceptual gap between horizontal and vertical communities to explain not just species composition and diversity, but also interactions themselves (Godoy et al. 2018; Marjakangas et al. 2022; Gravel et al. 2016; Ponisio et al. 2019). The stage therefore appears set for field-wide synthesis on processes that underlie species coexistence.
9.5.5 A final word on communities
With plant ecologists in the vanguard, the unification of functional approaches under the common currency provided by traits has had an impact that can hardly be overstated. In re-centering research on those specific characteristics that affect species fitness, functional community ecology has allowed scientists to identify and measure directly those variables that are thought to determine species coexistence and persistence. Because traits provide a basis for generalised predictions that are independent of taxonomy (McGill et al. 2006; Shipley 2016), they have opened the door to a generality in our understanding of the selective forces that shape communities that did not exist at the time of Lawton’s critique.
This is not to say that functional methods have ‘solved’ community ecology. Functional community ecology, which has grown so rapidly in the last decades, has yet to truly resolve several of its foundational tenets (Shipley 2016); some have even wondered whether the subfield has begun to ‘drift’ (Mittelbach and McGill 2019) or failed to live up to its promise (van der Plas et al. 2020). More to the point, current enthusiasm for the deterministic component of community assembly (‘rules of assembly’) denotes the influence of niche theory, but selective forces that operate on traits are just one of several processes that have been proposed as the singular explanation for diversity and composition. With Hubbell’s neutral theory of biodiversity (2001) and the advent of metacommunity ecology (Leibold et al. 2004), trait-neutral processes like drift and colonisation have also had their moment. What has emerged from these ideas are not universal laws that govern community dynamics in predictable ways, but a finite set of general high-level processes that fully specify the range of mechanisms about which community ecologists make predictions when they try to explain community structure (Vellend 2016).
9.6 So what?
Virtually all ecologists seek generality in their work. Statistical inference is based on the idea that what is true for a sample of cases is likely to generalise to a certain population of cases that have not been directly measured. This is formalised in scientific publications by statements about the range of conditions that our results extend or apply to.
Though individual ecological subdisciplines vary in scale and scope, they have this in common that they generally do not lend themselves to clean ‘yes or no’ answers. This is true for population dynamics, where both stochastic and deterministic factors interact to yield an endless variety of patterns. It is also true for macroecology, where even bold and robust trends like latitudinal diversity gradients are likely to be the result of both speciation and selective forces. As we have seen, this is most definitely the case of community ecology. McIntosh called this ecology’s ‘pluralism’ (1987), and it is a feature that ecology shares with several disciplines, including geology, evolutionary biology and all of the social sciences. But generality is not determined solely by system-level properties: it is a product both of the system and how we choose to study it (Fox 2019).
Thankfully, there are a number of ways to achieve generality (Cooper 2003; Fox 2019). The pattern-first approach has undoubtedly had resounding successes, not the least of which being the development of metabolic theory to explain robust scaling laws that had puzzled ecologists for decades. As we have shown, however, such an approach reaches its limits in cases where contingency and complexity are high and mechanisms are confounded.
The key to understanding the post-Lawton era of community ecology, and what will drive its future advance, is the move away from a pattern-based heuristic approach to explaining community patterns to a theoretically informed process-based perspective that relies on predictions and the evaluation of multiple competing or interacting mechanisms. Questions like ‘how does disturbance influence community diversity?’ should not rely on a unique and idiosyncratic hypothesis, but rather, on how a finite set of discrete high-level processes - immigration, drift and speciation, and finally selection (Vellend 2016) - combine to explain how communities change in response to disturbance.
This is not the first time that in the search for generality, Ecology has undergone a rapid shift towards more mechanistic approaches. With some fanfare, the rapid development of mathematical ecology through the 1980s came with the hope that mathematical models involving just one or two key processes would provide the firm basis that ecology needed. Throughout this chapter, we have intentionally remained vague on the question of whether the recent shift to trait-based approaches in community ecology constitutes a true Kuhnian paradigm shift. Writing in 2002, Paine argued that, because of its pluralism, ecology is unlikely to undergo changes that are quite so radical. After all, whether it is mathematical ecology, niche theory, neutral theory, metabolic theory or metacommunity theory, none of these advances have achieved quite the level of generality that they initially aspired to. Yet, all of them have been fully integrated into the ecological canon and now contribute to our understanding of ecological systems.
Whether the goal of ecology is to understand and predict the abundance and distribution of species, or how energy flows through the biotic and abiotic components of the ecosystem, it is unhelpful to declare that generality is impossible, or that ecologists should be satisfied with less of it. Achieving generality is, at least partially, under our control. Ecologists should continuously evaluate new methods and approaches critically, based on how much generality they help us achieve. It is our view that process-first approaches have propelled community ecology forward, a subdiscipline notoriously plagued with context dependence and complexity. We also believe that such approaches still have vast untapped potential, though this comes with significant (but bracing) challenges. Like opportunistic tinkerers, however, we will be keeping our eyes open for diminishing returns and on the lookout for the next idea that might take us even further.
9.7 References
Adler, P. B., J. HilleRisLambers, and J. M. Levine. 2007. A niche for neutrality. Ecology Letters 10:95–104. doi:10.1111/j.1461-0248.2006.00996.x
Allouche, O., M. Kalyuzhny, G. Moreno-Rueda, et al. 2012. Area–heterogeneity tradeoff and the diversity of ecological communities. Proceedings of the National Academy of Sciences of the United States of America 109:17495–17500. doi:10.1073/pnas.1208652109
Arrhenius, O. 1921. Species and area. Journal of Ecology 9:95–99. doi:10.2307/2255763
Bartley, T. J., K. S. McCann, C. Bieg, et al. 2019. Food web rewiring in a changing world. Nature Ecology and Evolution 3:345–354. doi:10.1038/s41559-018-0772-3
Bässler, C., M. W. Cadotte, B. Beudert, et al. 2016. Contrasting patterns of lichen functional diversity and species richness across an elevation gradient. Ecography 39:689–698. doi:10.1111/ecog.01789
Blowes, S. A., S. R. Supp, L. H. Antão, A. Bates, et al. 2019. The geography of biodiversity change in marine and terrestrial assemblages. Science 366:339–345. doi:10.1126/science.aaw1620.
Braun-Blanquet, J. 1932. Plant sociology. The study of plant communities. First edn. New York: McGraw-Hill.
Brown, J. H., J. F. Gillooly, A. P. Allen, et al. 2004. Toward a metabolic theory of ecology. Ecology 85:1771–1789.
Cadotte, M. W. 2007. Competition–colonization trade-offs and disturbance effects at multiple scales. Ecology 88:823–829. doi:10.1890/06-1117
Cadotte, M. W., C. H. Albert, and S. C. Walker. 2013. The ecology of differences: Assessing community assembly with trait and evolutionary distances’. Ecology Letters 16: 1234–1244. doi:10.1111/ele.12161
Cadotte, M. W., M. Carboni, X. Si, and S. Tatsumi. 2019. Do traits and phylogeny support congruent community diversity patterns and assembly inferences? Journal of Ecology 107:2065–2077. doi:10.1111/1365-2745.13247
Cadotte, M. W., K. Carscadden, and N. Mirotchnick. 2011. Beyond species: Functional diversity and the maintenance of ecological processes and services. Journal of Applied Ecology 48:1079–1087. doi:10.1111/j.1365-2664.2011.02048.x
Cadotte, M. W., T. J. Davies, J. Regetz, et al.. 2010. Phylogenetic diversity metrics for ecological communities: Integrating species richness, abundance and evolutionary history. Ecology Letters 13:96–105. doi:10.1111/j.1461-0248.2009.01405.x
Cadotte, M. W., and C. M. Tucker. 2017. Should environmental filtering be abandoned? Trends in Ecology and Evolution 32:429–437. doi:10.1016/j.tree.2017.03.004
Cardou, F., I. Aubin, A. Bergeron, and B. Shipley. 2020. Functional markers to predict forest ecosystem properties along a rural‐to‐urban gradient. Journal of Vegetation Science 31:416–428. doi:10.1111/jvs.12855
Catford, J. A., J. R. U. Wilson, P. Pyšek, et al. 2022. Addressing context dependence in ecology. Trends in Ecology and Evolution 37:158–170. doi:10.1016/j.tree.2021.09.007
Chao, A., C.-H. Chiu, and L. Jost. 2014. Unifying species diversity, phylogenetic diversity, functional diversity, and related similarity and differentiation measures through Hill numbers’. Annual Review of Ecology, Evolution, and Systematics 45:297–324. doi:10.1146/annurev-ecolsys-120213-091540
Chave, J. 2004. Neutral theory and community ecology. Ecology Letters 7:241–253. doi:10.1111/j.1461-0248.2003.00566.x
Chesson, P. 2000. General theory of competitive coexistence in spatially-varying environments’. Theoretical Population Biology 58: 211–237. doi:10.1006/tpbi.2000.1486
Clements, F. E. 1916. Plant succession: An analysis of the development of vegetation. Washington D. C.: Carnegie Institute of Washington.
Connell, J. H. 1978. Diversity in tropical rain forests and coral reefs. Science 199: 1302–1310.
Cooper, G. J. 2003. The pursuit of ecological generality. In The science of the struggle for existence: On the foundations of ecology, 1st edn, 96-127. Cambridge: Cambridge University Press. doi:10.1017/CBO9780511720154.
Delhey, K.. 2019. A review of Gloger’s rule, an ecogeographical rule of colour: Definitions, interpretations and evidence. Biological Reviews 94:1294–1316. doi:10.1111/brv.12503
Dornelas, M., N. J. Gotelli, B. McGill, et al. 2014. Assemblage time series reveal biodiversity change but not systematic loss. Science 344:296–299. doi:10.1126/science.1248484
Drude, O. 1896. Manual de geographie botanique. Paris: Librairie Des Sciences Naturelles.
Elton, C. S. 1927. Animal ecology. New York: Macmillan.
Forbes, S. A. 1887. The lake as a microcosm. Bulletin of the Peoria Scientific Association, 77–87.
Fox, J. W. 2019. The many roads to generality in ecology. Philosophical Topics 47:83–104. doi:10.5840/philtopics20194715
Garnier, E., J. Cortez, G. Billès, et al. 2004. Plant functional markers capture ecosystem properties during secondary succession. Ecology 85:2630–2637. doi:10.1890/03-0799
Gause, G. F. 1934. Experimental analysis of Vito Volterra’s mathematical theory of the struggle for existence. Science 79:16–17.
Gleason, H. A. 1926. The individualistic concept of the plant association. Bulletin of the Torrey Botanical Club 53:7–26. doi:10.2307/2479933
Godfrey-Smith, P. 2003. Theory and reality: An introduction to the philosophy of science. Chicago: University of Chicago Press.
Godoy, O., I. Bartomeus, R. P. Rohr, and S. Saavedra. 2018. Towards the integration of niche and network theories. Trends in Ecology and Evolution 33:287–300. doi:10.1016/j.tree.2018.01.007
Gravel, D., C. Albouy, and W. Thuiller. 2016. The meaning of functional trait composition of food webs for ecosystem functioning’. Philosophical Transactions of the Royal Society B: Biological Sciences 371:20150268. doi:10.1098/rstb.2015.0268
Grime, J. P. 1977. Evidence for the existence of three primary strategies in plants and its relevance to ecological and evolutionary theory. The American Naturalist 111:1169–1194. doi:10.1086/283244
Grime, J. P. 1997. Biodiversity and ecosystem function: The debate deepens. Science 277:1260–1261. doi:10.1126/science.277.5330.1260
Grime, J. P. 1998. Benefits of plant diversity to ecosystems: Immediate, filter and founder effects. Journal of Ecology 86:902–910. doi:10.1046/j.1365-2745.1998.00306.x
Hector, A., B. Schmid, C. Beierkuhnlein, et al. 1999. Plant diversity and productivity experiments in European grasslands. Science 286:1123–1127. doi:10.1126/science.286.5442.1123
Helmus, M. R., W. Keller, M. J. Paterson, et al. 2010. Communities contain closely related species during ecosystem disturbance. Ecology Letters 13:162–174. doi:10.1111/j.1461-0248.2009.01411.x.
Hobbie, S. E. 1992. Effects of plant species on nutrient cycling. Trends in Ecology and Evolution 7:336–339. doi:10.1016/0169-5347(92)90126-V
Hooper, D. U., E. C. Adair, B. J. Cardinale, et al. 2012. A global synthesis reveals biodiversity loss as a major driver of ecosystem change’. Nature 486:105–108. doi:10.1038/nature11118
Hooper, D. U., F. S. Chapin, J. J. Ewel, et al. 2005. Effects of biodiversity on ecosystem functioning: A consensus of current knowledge. Ecological Monographs 75:3–35. doi:org/10.1890/04-0922
Hubbell, S. P. 2001. The unified neutral theory of biodiversity and Biogeography. Princeton: Princeton University Press.
Huston, M. A. 1997. Hidden treatments in ecological experiments: Re-evaluating the ecosystem function of biodiversity. Oecologia 110:449–460. doi:10.1007/s004420050180
Hutchinson, G. E. 1978. An introduction to population ecology. New Haven: Yale University Press.
Keddy, P. A. 1992. Assembly and response rules: Two goals for predictive community ecology. Journal of Vegetation Science 3:157–164. doi:10.2307/3235676
Kraft, N. J. B., P. B. Adler, O. Godoy, et al. 2015a. Community assembly, coexistence and the environmental filtering metaphor. Functional Ecology 29:592–599. doi:10.1111/1365-2435.12345
Kraft, N. J. B., O. Godoy, and J. M. Levine. 2015b. Plant functional traits and the multidimensional nature of species coexistence. Proceedings of the National Academy of Sciences of the United States of America 112:797–802. doi:10.1073/pnas.1413650112
Krebs, C. J. 2000. Ecology: The experimental analysis of distribution and abundance, 5th edn. San Francisco: Benjamin-Cummings Publishing Company.
Laughlin, D. C. 2014. Applying trait-based models to achieve functional targets for theory-driven ecological restoration. Ecology Letters 17:771–784. doi:10.1111/ele.12288
Laughlin, D. C., C. Joshi, P. M. van Bodegom, et al. 2012. A predictive model of community assembly that incorporates intraspecific trait variation. Ecology Letters 15: 1291–1299. doi:10.1111/j.1461-0248.2012.01852.x
Lavelle, P., E. Blanchart, A. Martin, et al. 1993. A hierarchical model for decomposition in terrestrial ecosystems: Application to soils of the humid tropics. Biotropica 25:130–150. doi:10.2307/2389178
Lavorel, S., and E. Garnier. 2002. Predicting changes in community composition and ecosystem functioning from plant traits: Revisiting the Holy Grail. Functional Ecology 16: 545–556. doi:10.1046/j.1365-2435.2002.00664.x
Lawton, J. H. 1999. Are there general laws in ecology? Oikos 84:177–192. doi:10.2307/3546712
Leibold, M. A., M. Holyoak, N. Mouquet, et al. 2004. The metacommunity concept: A framework for multi-scale community ecology. Ecology Letters 7:601–613. doi:10.1111/j.1461-0248.2004.00608.x
Li, S., M. W. Cadotte, S. J. Meiners, Z. Hua, et al. 2015. Species colonisation, not competitive exclusion, drives community overdispersion over long-term succession. Ecology Letters 18:964–973. doi:10.1111/ele.12476
Loreau, M., and A. Hector. 2001. Partitioning selection and complementarity in biodiversity experiments. Nature 412:72–76. doi:10.1038/35083573
Lotka, A. J. 1925. Elements of physical biology. Baltimore: Williams and Wilkins.
MacArthur, R. H. 1958. Population ecology of some warblers of northeastern coniferous forests. Ecology 39:599–619. doi:10.2307/1931600
MacArthur, R. H. 1972. Geographical ecology: Patterns in the distribution of species. Princeton: Princeton University Press.
MacArthur, R. H., and E. O. Wilson. 1967. The theory of island biogeography. Princeton: Princeton University Press.
MacDougall, A. S., B. Gilbert, and J. M. Levine. 2009. Plant invasions and the niche. Journal of Ecology 97:609–615. doi:10.1111/j.1365-2745.2009.01514.x
MacIvor, J. S., N. Sookhan, C. A. Arnillas, et al. 2018. Manipulating plant phylogenetic diversity for green roof ecosystem service delivery. Evolutionary Applications 11:2014–2024. doi:10.1111/eva.12703
Mackey, R. L, and D. J Currie. 2001. The diversity-disturbance relationship: Is it generally strong and peaked? Ecology 82:3479-3492.
Marjakangas, E.-L., G. Muñoz, S. Turney, et al. 2022. Trait-based inference of ecological network assembly: A conceptual framework and methodological toolbox. Ecological Monographs 92:e1502. doi:10.1002/ecm.1502
Mason, N. W. H., D. Mouillot, W. G. Lee, and J. B. Wilson. 2005. Functional richness, functional evenness and functional divergence: The primary components of functional diversity. Oikos 111:112–18. doi:10.1111/j.0030-1299.2005.13886.x
Mayfield, M. M., S. P. Bonser, J. W. Morgan, et al. 2010. What does species richness tell us about functional trait diversity? Predictions and evidence for responses of species and functional trait diversity to land-use change. Global Ecology and Biogeography 19:423–31. doi:10.1111/j.1466-8238.2010.00532.x
McGill, B. J. 2003. A test of the unified neutral theory of biodiversity. Nature 422:881–885. doi:10.1038/nature01583
McGill, B. J., B. J. Enquist, E. Weiher, and M. Westoby. 2006. Rebuilding community ecology from functional traits. Trends in Ecology and Evolution 21:178–185. doi:10.1016/j.tree.2006.02.002
McIntosh, R. P. 1986. The background of ecology: Concept and theory. Cambridge: Cambridge University Press.
Meiners, S. J., M. W. Cadotte, J. D. Fridley, et al. 2015. Is successional research nearing its climax? New approaches for understanding dynamic communities. Functional Ecology 29:154–164. doi:10.1111/1365-2435.12391
Mittelbach, G. G., and B. J. McGill. 2019. Community ecology, second edn. Oxford: Oxford University Press. doi:10.1093/oso/9780198835851.001.0001
Naeem, S., L. J. Thompson, S. P. Lawler, et al. 1994. Declining biodiversity can alter the performance of ecosystems. Nature 368:734–737. doi:10.1038/368734a0
Nicolson, M., and R. P. McIntosh. 2002. H. A. Gleason and the individualistic hypothesis revisited. The Bulletin of the Ecological Society of America 83:133–142.
Niiniluoto, I.. 2019. Scientific progress. In The Stanford encyclopedia of philosophy, ed. E. N. Zalta, Winter 2019 edn. Stanford: Metaphysics Research Lab, Stanford University. https://plato.stanford.edu/archives/win2019/entries/scientific-progress/ accessed 23 December 2022.
O’Brien, M. J., B. M. J. Engelbrecht, J. Joswig, et al. 2017. A synthesis of tree functional traits related to drought-induced mortality in forests across climatic zones. Journal of Applied Ecology 54:1669–1686. doi:10.1111/1365-2664.12874
Odum, E. P. 1953. Fundamentals of ecology. Philadelphia: W. B. Saunders.
Oxford University Press. 2022. Oxford English Dictionary. https://www.oed.com/ accessed 12 December 2022.
Pacala, S., and A. P. Kinzig. 2013. Introduction to theory and the common ecosystem model. In The functional consequences of biodiversity, eds S. Pacala, A. P. Kinzig, and G. D. Tilman, 169–174. Princeton: Princeton University Press. doi:10.1515/9781400847303.169
Peters, R. H. 1980. Useful concepts for predictive ecology. Synthese 43:257–269.
Peters, R. H. 1991. A critique for ecology. Cambridge: Cambridge University Press.
Plas, F. van der, T. Schröder-Georgi, A. Weigelt, et al. 2020. Plant traits alone are poor predictors of ecosystem properties and long-term ecosystem functioning. Nature Ecology and Evolution 4:1602–1611. doi:10.1038/S41559-020-01316-9
Ponisio, L. C., F. S. Valdovinos, K. T. Allhoff, et al. 2019. A network perspective for community assembly. Frontiers in Ecology and Evolution 7. doi:10.3389/fevo.2019.00103
Poorter, H., U. Niinemets, N. Ntagkas, et al. 2019. A meta-analysis of plant responses to light intensity for 70 traits ranging from molecules to whole plant performance. New Phytologist 223:1073–1105. doi:10.1111/nph.15754
Popper, K. 1959. The logic of scientific discovery. 2nd edn. London: Routledge. doi:10.4324/9780203994627
Preston, F. W. 1960. Time and space and the variation of species. Ecology 41:612–627. doi:10.2307/1931793
Ricklefs, R. 2008. Disintegration of the ecological community. The American Naturalist 172:741–750. doi:10.1086/593002
Ricklefs, R., and R. Relyea. 2018. Ecology: The economy of nature. New York: Macmillan.
Schröter, C., and O. Kirchner. 1896. Die vegetation des bodensees. Kommissionsverlag der Schriften der Vereins für Geschichte des Bodensees und seiner Umgebung von J. T. Stettner.
Seibold, S., M. W. Cadotte, J. S. MacIvor, et al. 2018. The necessity of multitrophic approaches in community ecology. Trends in Ecology and Evolution 33:754–764. doi:10.1016/j.tree.2018.07.001
Shipley, B. 2016. Cause and correlation in biology: A user’s guide to path analysis, structural equations and causal inference with R. Cambridge: Cambridge University Press.
Shrader-Frechette, K. 2001. Non-indigenous species and ecological explanation. Biology and Philosophy 16:507–519. doi:10.1023/A:1011953713083
Simberloff, D. 1980. A succession of paradigms in ecology: Essentialism to materialism and probabilism. Synthese 43:3–39. doi:10.1007/BF00413854
Sims, P. L., and J. S. Singh. 1978. The structure and function of ten western North American grasslands: III. Net primary production, turnover and efficiencies of energy capture and water use. Journal of Ecology 66:573–597. doi:10.2307/2259152
Smith, F. A., S. K. Lyons, S.K. M. Ernest, and J. H. Brown. 2008. Macroecology: More than the division of food and space among species on continents. Progress in Physical Geography: Earth and Environment 32:115–138. doi:10.1177/0309133308094425
Smith, T. M., and R. L. Smith. 2014. Elements of ecology. 9th edn. Boston: Pearson.
Spalding, V. M. 1909. Distribution and movements of desert plants. Washington: Carnegie Institution.
Spehn, E. M., A. Hector, J. Joshi, et al. 2005. Ecosystem effects of biodiversity manipulations in European grasslands. Ecological Monographs 75:37–63. doi:10.1890/03-4101
Stein, A., K. Gerstner, and H. Kreft. 2014. Environmental heterogeneity as a universal driver of species richness across taxa, biomes and spatial scales. Ecology Letters 17:866–880. doi:10.1111/ele.12277
Thienemann, A. 1918. Lebensgemeinschaft und lebensraum. Naturwissenschaftliche Wochenschrift 17:282–290.
Tilman, D., S. S. Kilham, and P. Kilham. 1982. Phytoplankton community ecology: The role of limiting nutrients. Annual Review of Ecology and Systematics 13:349–372.
Tilman, D., D. Wedin, and J. Knops. 1996. Productivity and sustainability influenced by biodiversity in grassland ecosystems. Nature 379:718–720. doi:10.1038/379718a0
Vellend, M. 2016. The theory of ecological communities. Princeton: Princeton University Press. doi:10.1515/9781400883790
Vellend, M., L. Baeten, I. H. Myers-Smith, et al. 2013. Global meta-analysis reveals no net change in local-scale plant biodiversity over time. Proceedings of the National Academy of Sciences of the United States of America 110:19456–19459. doi:10.1073/pnas.1312779110
Vitousek, P. M., and W. A. Reiners. 1975. Ecosystem succession and nutrient retention: A hypothesis. BioScience 25: 376–381. doi:10.2307/1297148
Volterra, V. 1931. Leçons sur la théorie mathématique de la lutte pour la vie. Paris: Gauthier-Villars et cie.
Wardle, D. A. 1999. Is ‘sampling effect’ a problem for experiments investigating biodiversity-ecosystem function relationships? Oikos 87:403–407. doi:10.2307/3546757
Warming, E. 1925. Oecology of plants: An introduction to the study of plant-communities. Oxford: Oxford University Press.
Webb, C. O. 2000. Exploring the phylogenetic structure of ecological communities: An example for rain forest trees. The American Naturalist 156: 145–55. doi:10.1086/303378
Weiher, E., and . A. Keddy. 1995. Assembly rules, null models, and trait dispersion: New questions from old patterns. Oikos 74:159–164. doi:10.2307/3545686
Westoby, M. 1998. A leaf-height-seed (LHS) plant ecology strategy scheme. Plant and Soil 199:213–227. doi:10.1023/A:1004327224729
Whittaker, R. H. 1956. Vegetation of the Great Smoky Mountains. Ecological Monographs 26:2–80. doi:10.2307/1943577
Whittaker, R. H. 1960. Vegetation of the Siskiyou Mountains, Oregon and California. Ecological Monographs 30:279–338. doi:10.2307/1943563