Chapter 4 Growth and Physiology
Prokaryotes grow via a process called binary fission, during which DNA is replicated and chromosomes are partitioned:
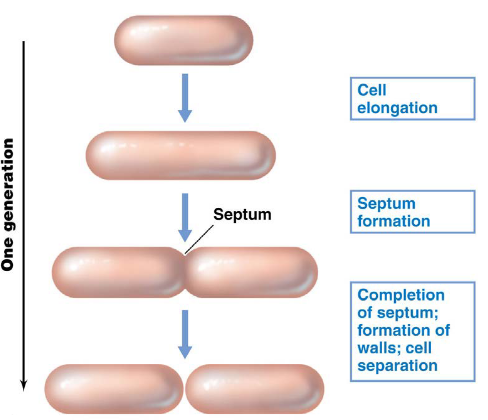
Figure 4.1: Binary Fission in a Hypothetical Bacterium
In the case of E. coli, it typically takes ~40 minutes to replicate DNA. 20 minutes after DNA replication, the bacterium then prepares for cellular division. One E. coli cell typically takes ~20 minutes to double due to the multiplicity of DNA replication.
4.1 Phases of Growth
The phases of growth can be summed up in the following graphic:
Figure 4.2: The Four Growth Phases
NB: A generation is the time it takes for a bacterial culture to double in its number of cells and mass!
4.1.1 The lag phase
In this phase, cells do not immediately divide when inoculated in fresh medium. Cells may also become larger to gear up for cell division.
It is worth noting that older cultures tend to have longer lag phases (generally speaking). Cells in this phase may also change the composition of the medium or environmental conditions to synthesize new enzymes.
4.1.2 The exponential phase
Here, cells undergo a constant, maximal growth rate under certain conditions.
The cell density N for a given time period t (given the initial cell density N0 and doubling time g) is shown in equation (4.1):
N=N02n=N02tg
Note that the actual determination of g is most easily done via a semi-log plot:
Figure 4.3: A Semi-Log Plot
Also, the easiest way to determine g is:
- Pick an interval on the y-axis that represents the doubling in biomass concentration.
- The corresponding interval from above on the x-axis is then g: the doubling time.
4.1.3 The stationary phase
Here, cells are alive, but not growing. Consequently, cells become smaller and have a reduced amount of ribosomes.
This phase can be initiated by a series of factors, including but not limited to:
- Substrate limitation
- O2 limitation due to high respiration rates and low O2 solubility
- Toxin production
This phase also induces genes that are necessary for survival and maintenance, hence also causing an increase in sporulation.
Cryptic growth is the phenomenon where the death rate is equivalent to the growth rate of the culture.
4.1.4 The death phase
Cell numbers decline rapidly in this phase and are unpredictable via mathematics. Cell death here may occasionally be accompanied by cell lysis.
It is important to note that while live cells can be observed by microscopy, viable colonies from transfer to agar plates may be lower. Hence, cells are not metabolically uniform in any given culture.
4.2 How do we Measure Microbe Growth?
There are several methods, each of which are listed and elaborated on in this section:
4.2.1 Direct counting
Out of all four methods, this is the fastest and also very accurate. Cells in this method are generally stained with a dye; dead cells are also counted in this method:
Figure 4.4: The Direct Count Method
4.2.2 Viable plate counts
This method assumes that a single cell will divide and, after a suitable incubation period, give rise to a colony forming unit (i.e., a CFU).
However, all media are selective - should you wish to use this method, you must know how to grow the bacteria!
Figure 4.5: The Viable Plate Count Method
4.3 Microbe Growth Conditions
Microbes grow in a variety of conditions. The conditions examined in BS2002 are shown below:
4.3.1 Air (or oxygen gas)
4.3.1.1 Aerobes
An aerobe is obligate if it requires O2 for growth.
Otherwise, an aerobe is a microaerophile if it grows with O2 levels that are below atmostpheric concentrations.
4.3.1.2 Anaerobes
An obligate anaerobe is a microbe that only grows in the absence of O2 via means of anaerobic respiration or fermentation.
An anaerobe is aerotolerant if it grows via fermentation, but is unable to utilize O2.
Lastly, an anaerobe is facultative if it is able to grow with O2, but also able to grow with inorganic e- acceptors and / or by fermentation.
4.3.2 Nutrient concentrations
The environment microbes live in is oligotrophic. The environment is complex, constantly changing, and often has low nutrient concentrations.
Hence, microbes must adapt to overlapping gradients of nutrients and environmental factors.
Leibig’s law of minimum states that the total biomass of an organism is determined by the nutrient at the lowest concentration.
Shelford’s law of tolerance states that a microbe, when placed outside of its environmental limits, will not grow regardless of the nutrient supply.
4.3.3 How do microbes adapt to low nutrients?
Microbes may become more competitive in nutrient capture and the use of available resources (e.g., efficient uptake systems).
Furthermore, there may also be morphological changes to increase surface area and the ability to absorb nutrients.
Bacteria may also develop certain mechanisms to sequester certain nutrients.
4.4 Environmental Effects on Microbial Growth
Extremophiles are microbes that grow under harsh conditions: conditions that would typically kill most organisms (including us humans).
In the majority of cases, extremophiles are prokaryotic, but even they have conditions under which they will not grow!
4.4.1 Environmental factors and ranges:
Some factors include:
- Temperature: from -36 degrees Celsius (Antarctic salt pools) to 122 degrees Celsius (hydrothermal vents)
- pH: from 0 to 11.5
- Osmotic effects: from pure water to 30% NaCl
- O2: with or without oxygen
- High pressure (the deep sea): barophiles
- Radiation: 3000000 to 6000000 rads (10000 rads is enough to kill a human!)
Note that no one organism survives over an entire range!
4.4.1.1 How does temperature affect growth?
Enzyme-catalyzed reactions are sensitive to temperature. A high temperature may cause enzymes to denature.
Furthermore, high temperatures may disrupt the cell membrane and cause DNA to denature.
Under freezing conditions, cell membranes become gel-like and there is no separation between phospholipid and protein domains. The structure of the cell membrane becomes homogenized:
Figure 4.7: Effects of Temperature on Microbial Survival
High quantitites of unsaturated fatty acids also lower the melting point; this also enables permease and membrane associated transport to function at low temperatures:
Figure 4.8: Optimal Growth Temperatures
4.4.1.2 pH
The internal pH of a microbe is maintained near neutrality (i.e., pH 7).
Acidophiles have an efficient consumption of H+ in the cytoplasm.
Alkaliphiles pump H+ into cell by Na+ / H+ antiporter.
4.4.1.3 Osmolarity
Water is the most important factor for controlling the growth of microorgranisms. In general, fungi are more tolerant to low water availability.
Compatible solutes are made in the cytoplasm to lower the availability of water inside the cell. These solutes are very polar and not toxic to the cell.
Bacteria make proline, glycine, betaine, and other related amino acids. Fungi make alcohol (e.g., glycerol) and sugars.
4.5 What Causes a Cell to Grow?
Proteins are located throughout the microorganism; DNA is present in the nucleoid, RNA in the cytoplasm (mRNA and tRNA), and ribosomes (rRNA).
Polysaccharides are present in the cell wall and some storage granules (i.e., poly β butyrate inclusions).
Lipids are present in the cell membrane, cell wall, and storage granules.
4.5.1 Nutrient requirements for bacteria
The following atoms: C, H, O, N, S, P are the building blocks of the macromolecules of life: proteins, lipids, nucleic acids, etc.
Potassium and sodium are important in membrane transport; calcium and magnesium are important cell wall polymer constituents and are also important in enzymatic functions.
4.5.2 Growth factors
Vitamins are components of enzymes, co-enzymes, and electron transport carriers.
Amino acids are needed for protein synthesis.
Purines and pyrimidines are required for nucleic acid synthesis.
Prototrophs grow on minimal media by synthesizing the required growth factors.
Auxotrophs are microorganisms that lack the ability to synthesize essential growth factors and requires it in the medium.
4.6 Growth Media
Growing media can come in different forms:
A defined media is a medium where all constituents are known.
In minimal media, all constituents are known, but there are no growth factors present.
Complex media have unknown compositions (i.e., they’re comprised of undefined substances); auxotrophs and prototrophs will grow on complex media.
Selective media favors the growth of particular microorganisms while inhibiting the growth of others.
Differential media is used to differentiate particular types of microbes based on growth and apperance (e.g., color differences). Test strips and kits can be used to test several activities at once, and growth on differential media can create visible clearing zone and use substrates that change color when products form.
4.7 Redox, Respiration, and Fermentation
Oxidation involves the loss of e-. An oxygen atom - the most effective e- acceptor due to their positive redox potential - can accept 2 e-.
Reduction involves the gain of e-. A hydrogen atom - the most efficient e- donors due to its negative redox potential - can donate one e-.
4.7.1 Directions of e- transfer
In exergonic reactions, e- transfers begin at molecules with more negative reduction potentials (E0) and ends at molecules with more positive E0. We also say that these reactions are catabolic.
However, endergonic reactions involves e- transfer in the other direction (i.e., from a more positive E0 to a more negative E0). We say that these reactions are anabolic.
4.7.2 Respiration versus fermentation
ATP can be formed via substrate-level phosphorylation (e.g., glycolysis) or oxidative phosphorylation (e.g., the e- transport chain and ATPase).
In fermentation, there are no terminal e- acceptors (i.e., the redox reaction occurs within the substrate). In aerobic respiration, O2 is the terminal e- acceptor; other inorganic molecules serve as the final e- acceptor for anaerobic respiration.
4.7.2.1 Glycolysis
This process is found in all domains of life and is the fastest way of providing quick energy from the metabolism of a glucose molecule into two pyruvate molecules. No e- transfer occurs here (not including the malate-aspartate shuttle).
However, ATP creation here is limited to two molecules of ATP per molecule of glucose. An excess of protons are also generated at the level of pyruvate.
4.7.3 Proton motive force
This was an idea first discovered by Peter Mitchell - a scientist who won the Nobel prize in 1971 for his chemiosmotic theory.
Mitchell’s theory was first described in mitochondria, but is also equally applicable to bacteria. This gradient is generated during the e- transport chain where e- are passed to multiple carriers inside the mitochondrial membranes and deposited against their concentration gradient.
Channels in the mitochondrial membrane have ATPase enzymes that capture energy as protons move down their concentration gradients:
4.8 Anabolism and Catabolism
4.8.1 Anabolism
Anabolism is the biosynthesis of complex molecules from simpler ones with the input of energy.
Close proximity and coupling of catabolic and anabolic pathways is important with limited resources; the rate of biosynthesis is approximately balanced by the rate of catabolism.
Different cofactors are also used for anabolism and catabolism: NADH in catabolism and NADPH in anabolism.
4.8.2 Catabolism
The catabolism of polysaccharides and proteins is widespread among all life forms. Catabolic reactions reduce macromolecules to monomeric units that are eventually metabolized for energy.
Polymers hydrolyzed by exoenzymes are excreted through the cell wall or extending from the periplasm (e.g., amylase of Bacillus).