Chapter 4 Forest Rockfall Control
Compared to other natural hazard processes, rockfall is a phenomenon that is difficult to predict because the process area is not clearly predefined. After an introduction to the process, this chapter describes the various mechanisms and limits of forests to minimize the risk of rockfall.
Gravity-induced fall processes of mostly regolith can be classified according to their size or volume and velocity, as shown in the following table.
Type | Diameter | Volume | Velocity | Comments |
---|---|---|---|---|
Rockfall (rocks) | <50cm<50cm | −− | <30m/s<30m/s | usually single stones per event |
Rockfall (blocks) | ≥50cm≥50cm | <102m3<102m3 | <30m/s<30m/s | usually single blocks per event |
Rock mass fall | −− | 102m3to106m3102m3to106m3 | 10−40m/s10−40m/s | The rock masses from large detachments (rockslides) mostly fragment very rapidly due to their high energies and then move down the valley as extremely fast flows of rocks (Preh 2020). |
Rock avalanche | −− | >106m3>106m3 | >40m/s>40m/s | The rock masses of large rockslides usually fragment very quickly due to the high energies involved and then move downhill as extremely fast streams of fragmented rock (Preh 2020). |
Here we focus exclusively on rockfall processes, which generally occur in the form of low intensity and high frequency events, enabling the high protective effect of forests. Rockfall occurs when stone fragments break loose and travel along a steep slope at speeds of 5 up to 40 m/s. However, in contrast to other natural hazards, rockfall is unpredictable due to various influencing factors such as geology, climate, weathering, vegetation and human activities. Nevertheless, it has been shown that rockfall often occurs in spring, as a result of frost and thaw and after heavy rainfall (L. Dorren 2003; Erismann and Abele 2001).
The effect of forests on rockfall is variable, depending on the location along the rockfall path. In general three different zones can be distinguished: source area, transition area and deposition area (Fig.:4.1).
![Three main rockfall process zones. Modified according to [@Dorren2007]](figures/rockfall_zones.png)
Figure 4.1: Three main rockfall process zones. Modified according to (L. Dorren et al. 2007)
4.1 Source area (triggering)
In the source area, triggering of rockfall might depend on:
Geological factors, especially tectonic activity, are key drivers of rockfall events. Tectonic forces can steepen hillslopes, making them more prone to rockfall. Earthquakes, whether large and infrequent or small and frequent, can trigger rockfalls by causing ground shaking that dislodges rocks. Additionally, tectonic stresses can cause the dilation of rock masses, leading to the formation and widening of joints and fractures. This process weakens the rock structure, making it more susceptible to failure. Weathering processes further exacerbate this by reducing the strength of the rock, increasing the likelihood of rockfall.
Weathering processes significantly contribute to the triggering of rockfall by altering the stability of rock masses. The opening of joints within rocks, often caused by physical or chemical weathering, creates pathways for further weakening. As weathering continues, the asperities—rough, uneven surfaces—of bedding planes and joints are worn down, reducing friction and cohesion between rock layers. Additionally, the weathering process can lead to the formation of clay-rich infills within joints, which absorb water and swell, further destabilizing the rock structure. These factors collectively weaken the rock, making it more susceptible to rockfall events
Human activities can significantly trigger rockfalls by altering the stability of slopes. The cutting of slopes for construction, road building, or mining often removes the natural support of rock masses, making them more prone to collapse. Blasting, commonly used in mining and construction, can also induce rockfalls by opening existing joints and creating new fractures within the rock. These activities disturb the natural balance of the slope, increasing the likelihood of rockfall events as the fractured rock loses cohesion and becomes unstable.
4.1.1 Forest effects within the source area
Vegetation in general and forests in particular play a complex role in rockfall triggering, with both positive and negative effects on slope stability. On the negative side, the roots of large trees can penetrate existing joints and bedding planes in bedrock, acting as wedges that pry the rock apart and increase the likelihood of rockfall.
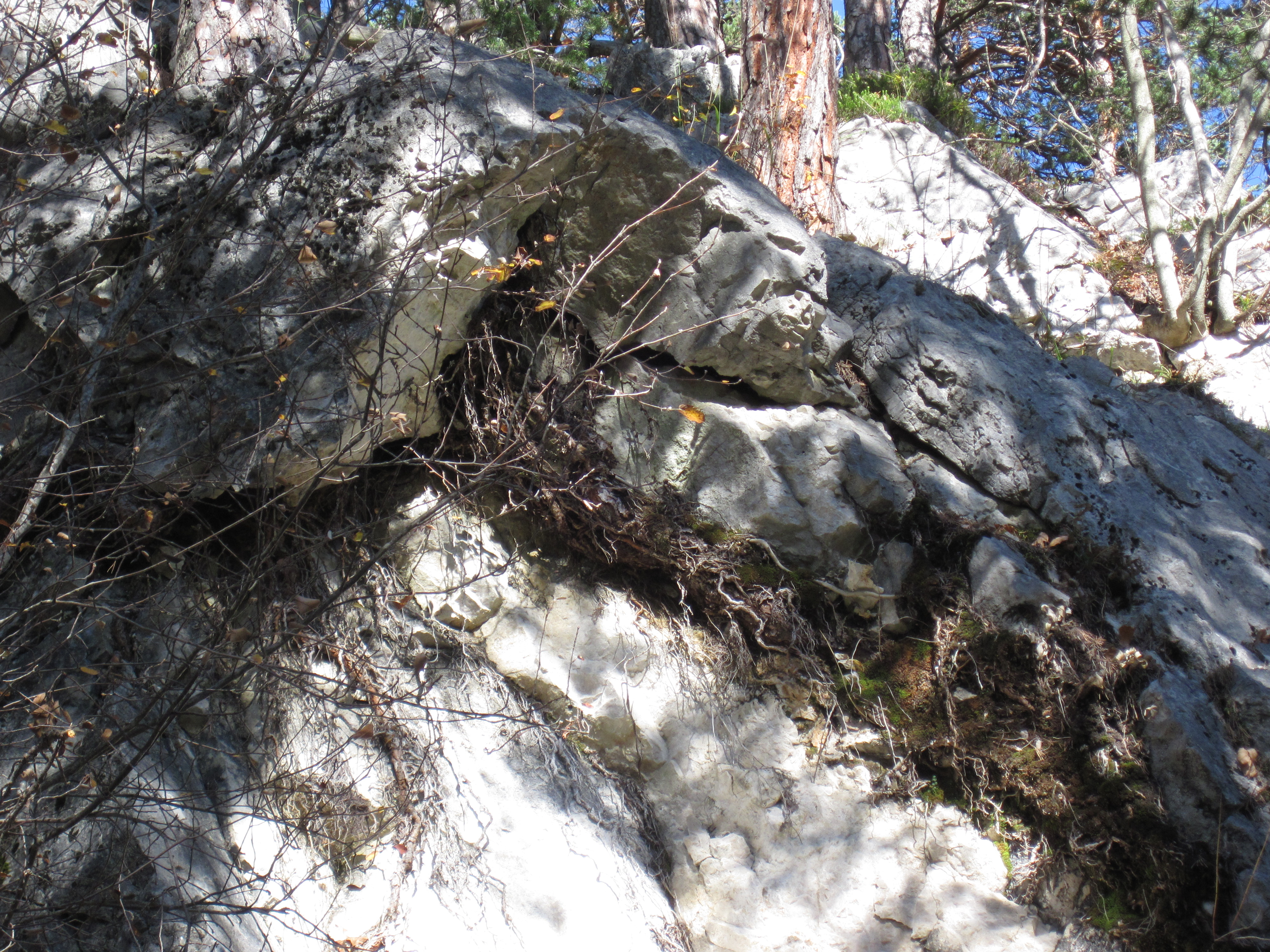
Figure 4.2: Negative effect of forest stands in a rockfall source area. Roots penetrating rock joins - increasing the likelihood of rockfall.
Additionally, movement of the tree stem caused by wind or snow can amplify this wedge effect, further destabilizing the rock. The presence of roots in the bedrock can also accelerate chemical weathering, weakening the rock and making it more prone to failure. However, forest can also have a stabilizing influence. Stable, standing trees can act as natural barriers, slowing down or even stopping falling rocks, particularly in steep areas where rockfall is more likely.
4.2 Transition area (motion)
In the rockfall process occurring within a transition zone, energy dissipation is governed by the transformation and distribution of potential and kinetic energy. Initially, the rock’s potential energy, dependent on its height and mass, converts into kinetic energy as it begins to fall. This kinetic energy is further divided into translational and rotational energy, where translational energy propels the rock downward while rotational energy causes it to spin. The extent of kinetic energy loss during the rockfall is influenced by several factors: the mass of the rock (with larger rocks retaining more energy and thus traveling farther), the slope inclination (steeper slopes lead to greater acceleration and energy), damping conditions (which can absorb energy and slow down the rock), and collisions with surface structures, which can significantly reduce energy through impact. The runout distance, or how far the rock travels, is therefore directly related to the rock’s mass and energy, as larger rocks with higher energy tend to cover longer distances. Additionally, the shape of the rock plays a crucial role in determining the type of movement it undergoes, such as falling, gliding, rolling or jumping. (Fig.:4.3).
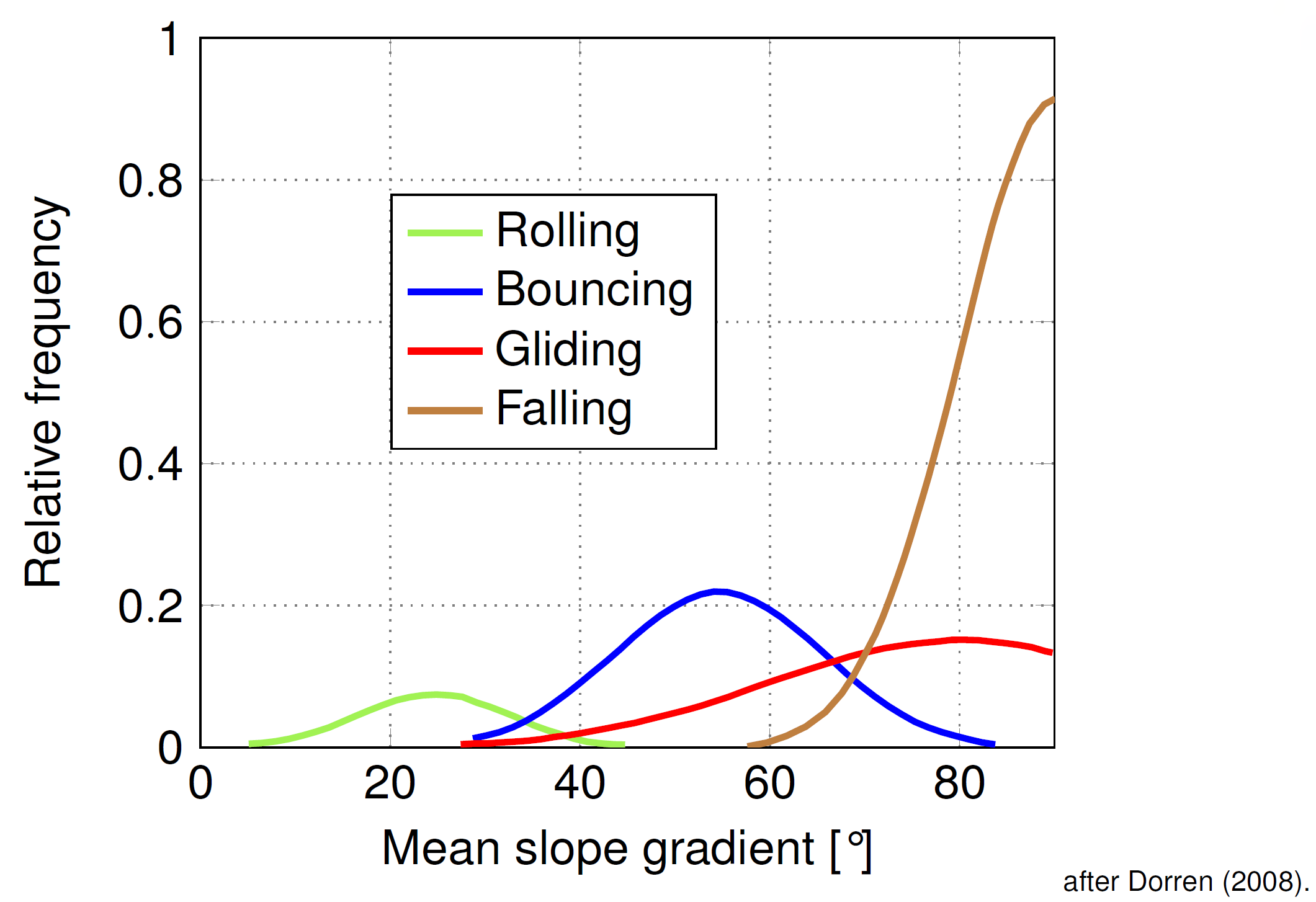
Figure 4.3: Distribution of motion depends on mean slope gradient.
Irregularly shaped rocks are more prone to bounce or tumble, leading to more chaotic and unpredictable movement paths, while smoother, more rounded rocks may glide or slide more uniformly. These variations in movement further affect the energy dissipation and ultimately influence how far and where the rock will come to rest. |
### Forest effects within the transit and deposit area {#se:rock_transit_for} |
In general, forest cover has the greatest influence on the occurrence of a rockfall event in the transit zone, where the fall heights and velocities of the rocks reach their maximum (Flageollet and Weber 1996; Leine et al. 2014; L. Dorren 2016; Yan et al. 2018). A forest reduces both, velocity and thus jump height of the falling rocks, as the energy of individual trees is dissipated. However, it has proven to be very difficult to quantify the total amount of energy absorbed, as a single tree can absorb energy in different ways (L. Dorren et al. 2007). This depends on the translational and rotational potential of the root system, the possible deformation of the oscillation of the tree trunk on impact and the local penetration of the rock at the impact site (Fig.: 4.4). |
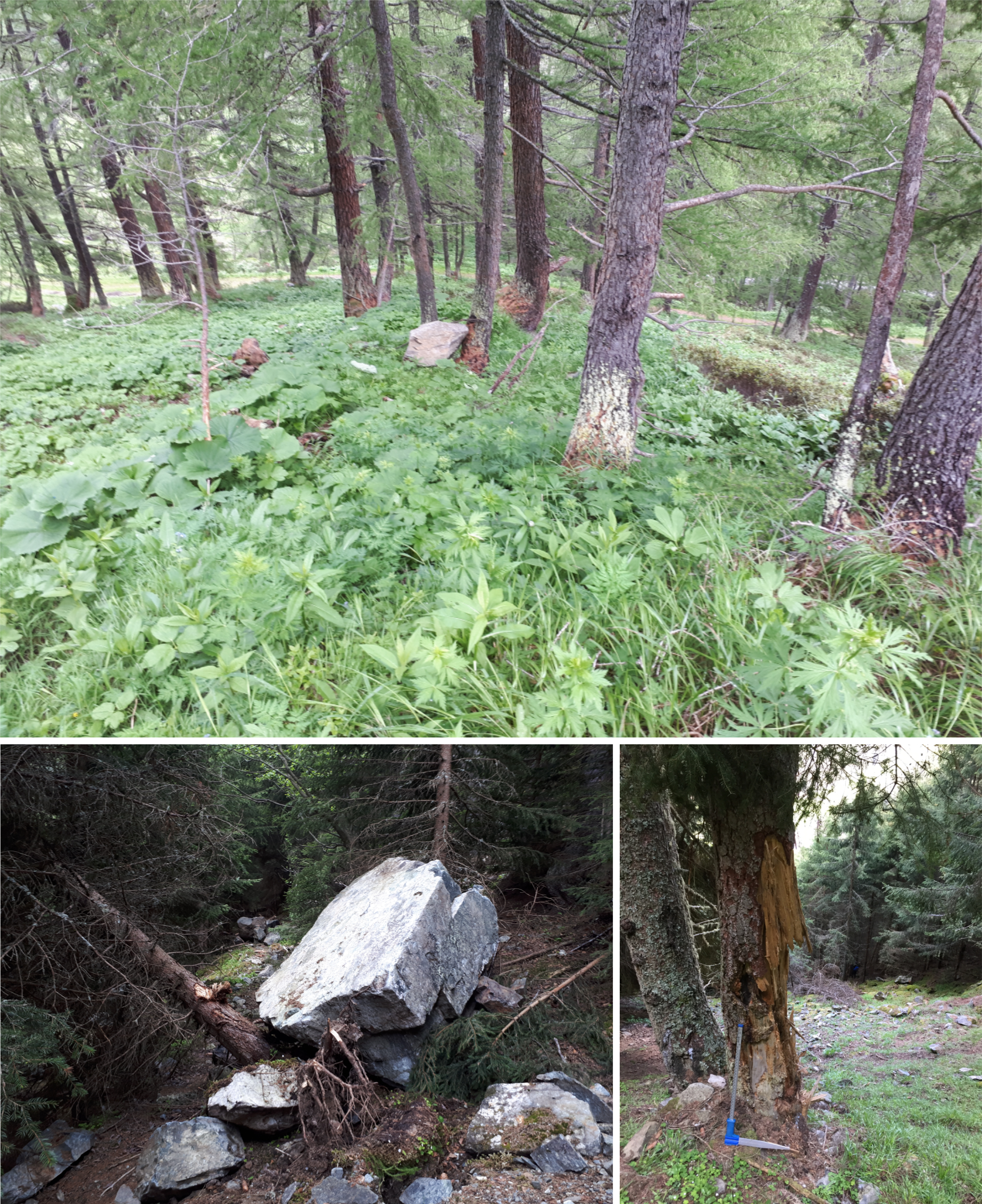
Figure 4.4: Effect of the forest on rockfall. (Photo: Lauss E.)
A general relationship between the diameter at breast height (DBH) and the range of energy that can be dissipated during a rockfall impact lower than 2m2m on the tree stem for broad-leaved and coniferous species is shown in Figure (4.5). |
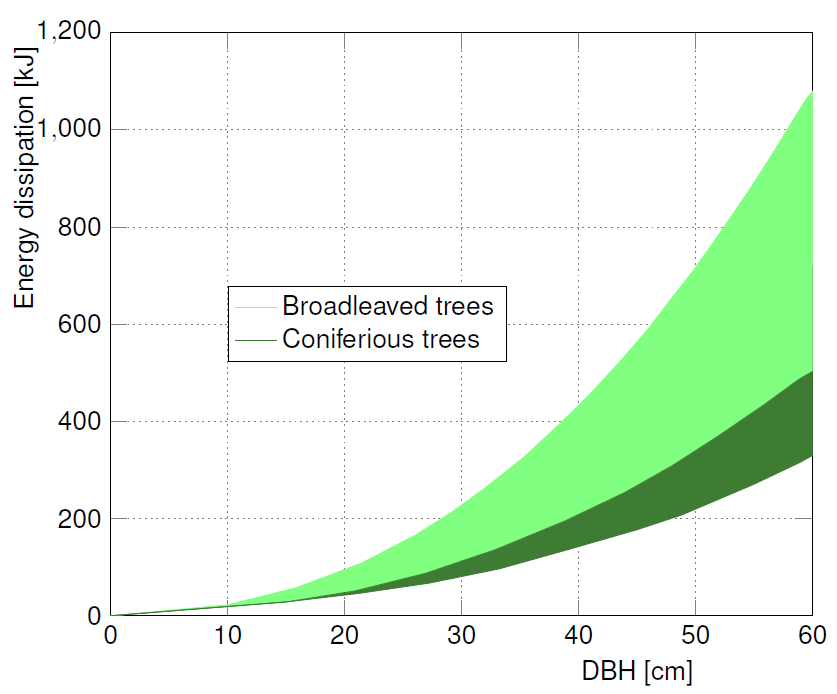
Figure 4.5: Difference between energy dissipation of coniferous and broadleaved tree species. Modified after L. Dorren et al. (2007)
A rockfall experiment with a 1m31m3 rock hitting a fir with 0.58m0.58m DBH. Experiments carried out by Frederic Berger and Luuk Dorren, 2001-2004 at Cemagref Grenoble, France. ***
An effect of forests on rockfall was first systematically analysed by Jahn (1988) who moved rocks downhill along forest-covered and unstocked slopes. He found about 60%60% of the stones in covered sections stopped as a result of tree contacts. Further, 3–10 times more stones were deposited in covered than in uncovered areas, and the kinetic energy of a stone’s mass center was reduced by about 80%80% if a tree was squarely hit. Figure (4.6 shows the velocities and run-out distances of the fastest stone and the average velocity of all stones that passed the non-forested area or the forested area.
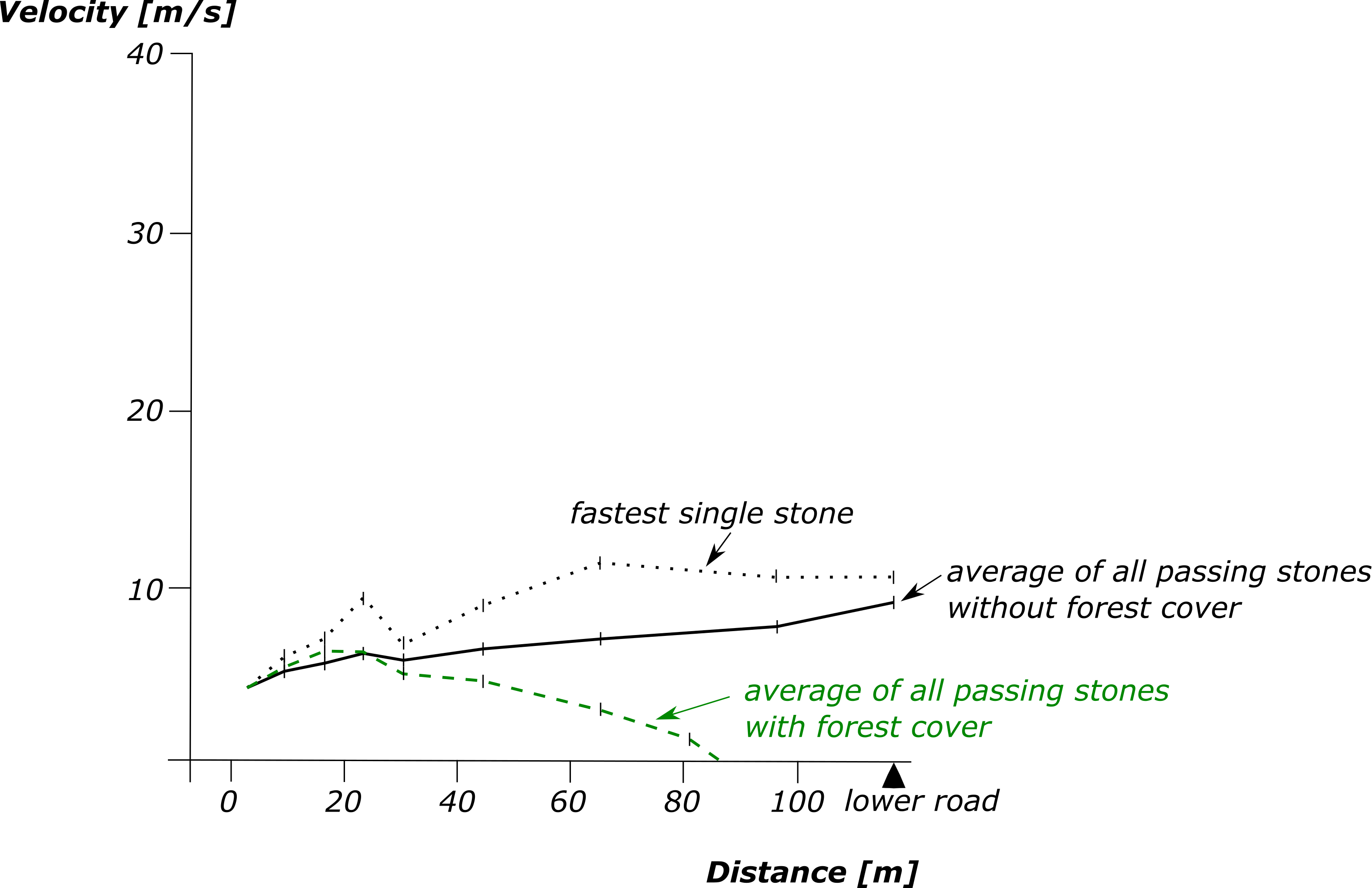
Figure 4.6: Different section velocities of stones passing forested and non-forested areas. After Jahn (1988)
The following table compares travelling velocities and rebound heights between a non-forested and a forested slope.
Rockfall parameters | Non-forested (n = 100) | Forested (n = 102) |
---|---|---|
Average translation velocity | 11m/s11m/s | 8m/s8m/s |
Average maximum translation velocity | 15.4m/s15.4m/s | 11.7m/s11.7m/s |
Maximum translation velocity | 30.6m/s30.6m/s | 24.2m/s24.2m/s |
Number of rocks stopped after 223.5 m (end of forested zone) | 55 | 6565 |
Mean rebound height | 1.5m1.5m | 1m1m |
Maximum rebound height | 8m8m | 2m2m |