Chapter 2 Understanding the Deep-Sea
Until relatively recently the deep-sea was thought to be a lifeless desert. The last thirty years of deep ocean exploration have transformed our understanding of the deep seas, deep-sea habitats and the diversity of marine organisms.
This chapter focuses on introducing the diversity of deep-sea marine habitats and marine organisms. It assumes no prior knowledge of the deep-sea but also seeks to bring readers more familiar with these issues up to date on the latest scientific research on deep-sea habitats, threats and issues. We begin with a brief overview of the legal context of debates on research on marine genetic resources in Areas Beyond National Jurisdiction before moving into discussions of specific habitats and threats.
2.0.1 The Legal Context
Two main legal instruments are relevant to marine genetic resources. In the case of marine genetic resources inside the territorial boundaries of states and Exclusive Economic Zones (EEZs), the United Nations Convention on Biological Diversity is an important instrument. However, for the marine environment as a whole and Areas Beyond National Jurisdiction (ABNJ) the main instrument is the United Nations Convention on the Law of the Sea (UNCLOS). The 1982 United Nations Convention on the Law of the Sea is the foundation of international law for the marine environment and has been ratified by 166 states.2 UNCLOS enshrines the principle of freedom of the high seas and sets out states’ rights and responsibilities with respect to issues such as state limits, navigation, Exclusive Economic Zones (EEZs), the continental shelf, deep-seabed mining, the environment, Marine Scientific Research (MSR) and transfer of technology.
Areas Beyond National Jurisdiction are divided into two categories having specific legal regimes. These are the water column, subject to the freedom of the high seas and addressing navigation, fishing and Marine Scientific Research (MSR) under Part II of UNCLOS [14], and the seabed in Areas Beyond National Jurisdiction, known as “the Area”, addressed under Part XI of UNCLOS [14].
UNCLOS is silent on the subject of marine genetic resources although Article 136 of UNCLOS establishes that the seabed and its resources are the common heritage of mankind [14,27]. However, it is important to note that these resources are described as “all solid, liquid and gaseous resources in situ in the Area at or beneath the seabed, including polymetallic nodules” (Article 133) [14,27]. In the early years of debate on marine genetic resources under UNCLOS the concept of common heritage of humankind sparked considerable debate about whether marine genetic resources could, or should, be considered to legally fall under the concept of common heritage [14,15,18]. This chapter is not concerned with the legal interpretation of UNCLOS or recent debates on marine genetic resources. A growing literature provides guidance on this topic [3,19,20,26]. Instead, our purpose is to consider available data on biodiversity, habitats and threats in Areas Beyond National Jurisdiction. For ease of use we use the generic term deep-sea to refer to Areas Beyond National Jurisdiction. Our use of this term encompasses both the water column (the High Seas) and the seabed (the Area) in Areas Beyond National Jurisdiction. Figure 2.1 displays the areas covered in this chapter and the wider report.
It is important to note that the limits of Exclusive Economic Zones may be a subject of disputes between states. Given that the limits of particular EEZs are not an issue addressed by this report we use the term “the EEZ” in the singular throughout this report and display the EEZ in maps simply as a global outline based on data from the Global Bathymetric Chart of the Oceans (GEBCO).
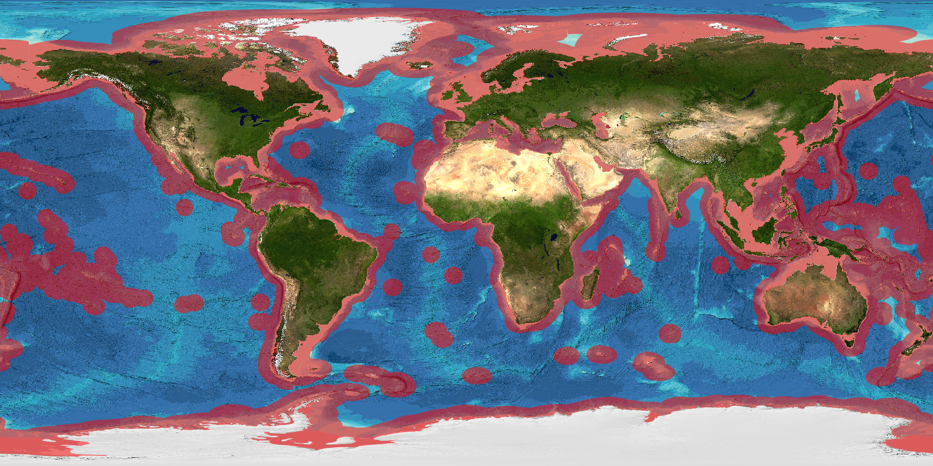
Figure 2.1: The EEZ extends 200 nautical miles beyond the coast (red shaded area). ABNJ encompasses the high seas and the seabed (the Area). (Source: GEBCO)
2.0.2 Deep-Sea Habitats
The scientific exploration of the high seas began in earnest with the expedition of HMS Challenger between 1872 and 1876 when the ship sailed 69,000 miles taking samples and readings from all of the world’s oceans apart from the Indian Ocean [28]. Yet today, 140 years later, we have still only explored a tiny fraction of this vast area. Understanding the deep oceans has proved to be a great challenge requiring new technological developments to enable each exploratory step forward, and even today new discoveries are made with almost every scientific cruise. The complex interactions of ecology, geomorphology, ongoing geologic processes, hydro-dynamics, light levels, chemical composition, temperature and pressure as well as remoteness and inaccessibility mean that the deep oceans remain the last great wilderness on the planet.
The deep-sea begins at about 200m depth at the break of the continental shelf. At the time UNCLOS was agreed, the deep-sea was considered to have low biodiversity, no seasonality and an unvarying environment. This began to change in the late 1970s and 1980s when habitats such as hydrothermal vents, cold seeps and chemosynthetic ecosystems were discovered. Prior to these discoveries, the deep-sea outside of national jurisdiction was often perceived as being of utility either for waste disposal or as a source of potential mineral wealth. While understanding has grown of the importance of biodiversity in the deep-sea there is significant concern within the scientific literature and among participants in our expert Delphi study that exploitation is advancing faster than the acquisition of scientific knowledge. With this exploitation comes the risk of damaging the deep sea before we have adequate knowledge of how best to protect it. In particular the scientific literature and our expert Delphi study participants highlight the risks posed by commercial fishing, mining and pollution that far outweigh the dangers of marine scientific research.
Scientific knowledge and understanding of biodiversity in the deep-sea now extends from life near the surface of the oceans through the water column, to the sea-bed and deep into ocean sediment and the oceanic crust that makes up the deep biosphere. In debates on access to genetic resources and benefit sharing, attention has tended to focus on the most charismatic of the deep-sea habitats - hydrothermal vents. However, it is important to gain a wider view of the full spectrum of deep-sea habitats in considering possible options for any implementing agreement under UNCLOS. For ease of explanation we begin with the water column.
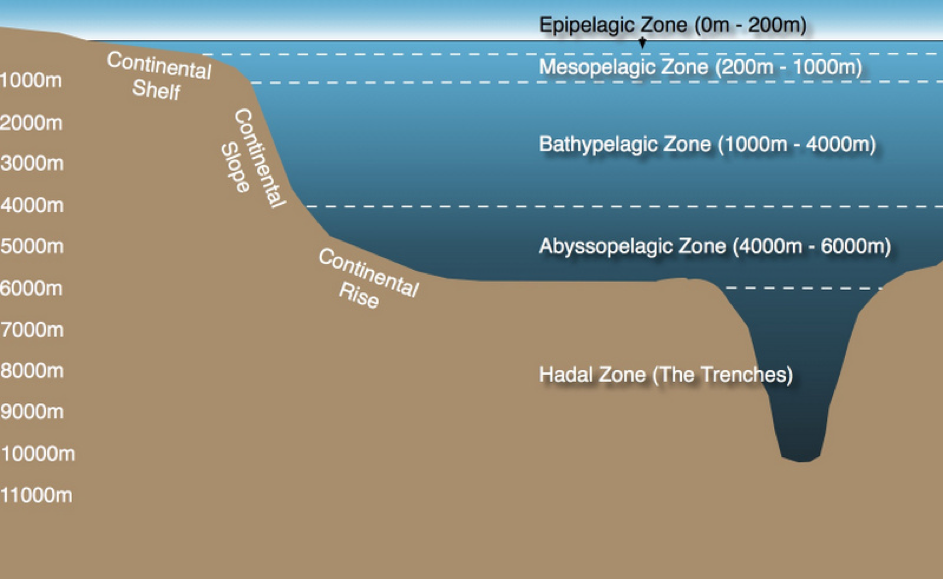
Figure 2.2: The Pelagic Zones
The Water Column
The water column comprises all the open ocean waters and is divided into sections known as pelagic zones at different depths (Figure 2.2). In the context of the Census of Marine Life a team of researchers reviewed 7 million georeferenced marine records from the Ocean Biogeographic Information System to assess current scientific coverage of organisms in the water column [29]. In a paper entitled ‘Biodiversity’s Big Wet Secret’, the research team revealed the deep pelagic ocean below 200 metres to be the most under-represented sphere in global marine biology databases [29]. The research demonstrated that the majority of our knowledge of marine biodiversity is derived from shallow seas and that our knowledge of other regions of the ocean is often disproportionate to its geographical area. For instance, “more than 50% of all records come from the continental shelf which constitutes 10% of the ocean whereas less than 10% of records come from the abyssal plain which constitutes 50% of the ocean area” ([29]: 3). Figure 2.3 demonstrates that most records have come from a narrow band of water at the ocean surface or at the sea bed and that there is a paucity of data relating to the pelagic “mid water” zone. As we discuss in the next chapter, this concentration of research produces major gaps in our knowledge and understanding of biodiversity that need to be filled.
Historically, it was believed that the pelagic region was lower in biomass than the surface and floor of the ocean. However, it has recently been argued that it is lack of sampling and limitations in sampling techniques that have created this void in knowledge and understanding rather than an absence of biodiversity. Long term in situ sampling is beginning to reveal the true extent of biodiversity in the water column [29]. However, participants in the expert Delphi study highlight that the middle of the water column represents a neglected area of research activity. The same can also be said of the second dominant area of the oceans: the abyssal plain.
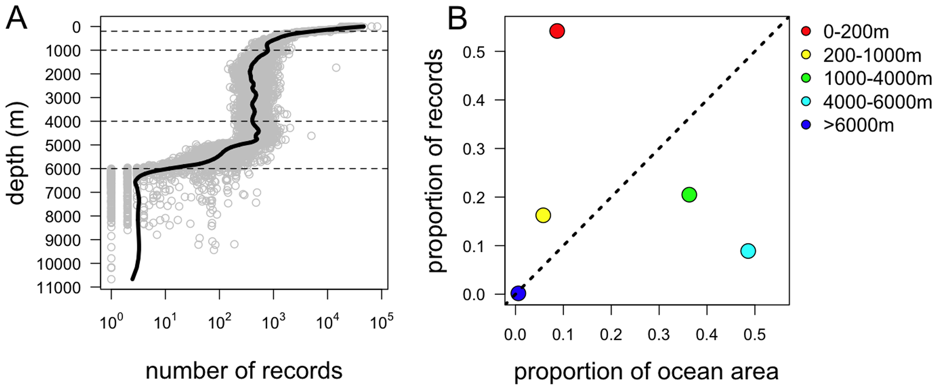
Figure 2.3: The Depth Distribution of OBIS Records of Global Marine Biodiversity. doi:10.1371/journal.pone.0010223.g001.3
The Abyssal Plain
Abyssal plains are vast areas of flat sediment covered seabed stretching between continental margins and mid-ocean ridge systems. They are usually found at depths of 3000–6000 m and cover approximately half of the Earth’s surface [30]. Abyssal plains result from the blanketing of the basalts of the oceanic crust by fine-grained sediments. Due to their great area these sediments are quantitatively important for the global carbon cycle and the primary ecosystem services provided by the deep-sea (see below) [31].
The sheer sizes of the abyssal plains are the greatest barriers to their exploration. In early studies of abyssal infauna (animals living in marine sediment), regardless of location, roughly 90% of infaunal species collected were new to science [30]. CeDAMar (the Census of the Diversity of Abyssal Marine Life) was designed to sample previously under-explored areas through a programme of expeditions. In summarising results from these expeditions, Ebbe et al. 2010 put forward two main observations: (1) extreme is normal; (2) rare is common. In other words, what we anthropocentrically consider extreme conditions are in fact normal conditions and highly hospitable for a range of organisms, and the abundance of species thus far identified is actually based on a relatively small number of records. Thus, based on our current knowledge, nearly all species from the abyss can be considered rare and there are no species dominating any sampled areas [30]. These general results must, however, be viewed in the context of the vast area of the abyss. Species currently considered rare or endemic to a particular location, may simply not have been found elsewhere yet [30].
Species abundance on the abyssal plains was found to be directly related to the availability of food in the form of ‘marine snow’ (organic matter descending through the water column). Researchers are concerned about how potential changes to this primary organic matter will impact on benthic (seafloor) communities. A 24 year project measuring changes in food supply and utilisation by benthic communities at 4,000 m in the north east Pacific has recently ended, revealing significant connectivity between changing surface ocean conditions and benthic communities which ultimately impact on the global carbon cycle [32]. The amount of marine snow has been shown to vary according to seasonal changes in shallow water biomass (such as surface diatoms) and that organic material incorporated into abyssal sediments provides a larder for times of reduced marine snowfall. It is uncertain whether the amount of available primary organic matter is stable and how climate change might affect this [32]. Manganese nodules are small polymetallic nodules of iron and manganese hydroxides found scattered in large fields across the abyssal plain [30]. These rocky nodules provide what is often the only hard substratum in the sediment dominated plains and thus add habitat variety enabling sessile fauna to gain a foothold, thereby increasing ecological complexity [30]. Manganese nodules are a long-standing focus of potential for sea floor mining activity [33,34].
Seafloor and subseafloor sediments
Microbes dominate life in seafloor sediments and measures of abundance of microbial life typically use cell counts as the primary measure. As reported by Orcutt et al., (2013) it is generally assumed that metabolic rates in the deep biosphere are low, yet potentially capable of influencing important global biogeochemical cycles (involving elements such as C, H, O, N, Fe, Mn and S). For example, drawing on the work of Reeburgh et al., Orcutt at al. 2013 highlight that “sedimentary microbial processes account for oxidation of 95% of the methane that exists in marine sediment, reducing the amount of methane flux to the water column” [35]. This indicates the important role played by microorganisms in this biosphere.
Studies of microbial life in subseafloor sediment have found strong correlations between the availability of organic matter and cell abundance, with buried organic matter inferred to be the primary source of energy for microbes in most subseafloor sediment [36]. Using measurements of the concentration of intact phospholipids (IPLs) as a proxy for microbial biomass, cell abundance was found to be greatest at continental margins, where approximately 33% of total subseafloor cells live in just 7% of the total oceanic area, and lowest in mid ocean gyres (large systems of rotating currents) where 10% of the total cells live in about 42% of the total oceanic area [36]. Estimates suggest a subseafloor microbial abundance, which is roughly equal to that in seawater and that in soil. It also approximates with the lower estimates of microbial abundance in the terrestrial subsurface [36].
In seeking to distinguish types of microbial life in this realm there is an ongoing debate about whether archaea or bacteria dominate life in sediments. Studies have generally found archaea to be more dominant than bacteria in subseafloor sediments [37,38]. However, more recently, Xie et al. 2013 concluded that a substantial portion of archaeal IPLs found in subseafloor sediments are probably fossil products of past cell generations and that previous estimates of microbial life based on IPLs were probably too high [39]. Xie’s results cast doubt on Kallmeyer’s estimates and highlight the challenges of distinguishing between bio and geo molecules. The results also offer the possibility that life in the subseafloor sediment is dominated by bacteria [39].
Further studies have sought to distinguish the types of organisms present in sediments from different sites on the deep seafloor, notably organic falls (predominantly whale carcasses fallen to the seabed on migration routes), hydrothermal vents and cold seeps [40]. Most studies at these sites have focused on macrofauna. Each of these three soft sediment habitats is fuelled by oxidation of reduced chemicals. Results show that these environments are heterogeneous, sharing dominant taxa but being distinct at family level. The authors conclude that: “although the macrofaunal structure (family level) of vents, seeps and falls exhibit some commonalities such as low diversity and high dominance of a few polychaete (‘bristle worm’) taxa, community level analyses reveal strong differences. These differences are due to stresses (e.g. high temp, high sulfides, low oxygen) and poorly known depth trends, biogeographic isolation and evolutionary divergence” [40].
![Figure 2.4: Vertical section of the seabed and seafloor structures. Adapted from [41].](Images/vertical_section_of_seabed_seafloor.png)
Figure 2.4: Vertical section of the seabed and seafloor structures. Adapted from [41].
Oceanic Crust:
If there exists this deep, hot biosphere, it will become a central item in the discussion of many, or indeed most, branches of the Earth sciences. How much of the biological imprint of material in the sediments is due to surface life and how much to life at depth?
(Gold 1992, cited in Orcutt et al., 2013)
Beneath the seafloor lie habitats that may prove to be of major importance for their unique biodiversity. Slow progress in ocean drilling techniques has hampered ability to sample directly from the deep subsurface. However, the 1977 discovery of hydrothermal venting at the Galapagos mid-ocean ridge (MOR) spreading centres drew significant scientific attention to hydrothermal systems which came to be considered “windows to a subsurface biosphere” [42,43]. These hydrothermal systems, and the ability of their microbiology to survive extreme conditions, have remained a focus for exploration of novel enzymes, genes and microorganisms. In considering these systems as windows where volcanic activity ‘throws up’ microbial life from below, scientists have used activity at hydrothermal sites to estimate life in the underlying deep biosphere. Hydrothermal vents have received significant attention in debates on access and benefit-sharing in Areas Beyond National Jurisdiction. However, the role of hydrothermal vents as windows into the deep biosphere has tended to be lost in discussions of these charismatic locations and their unusual fauna. This is unfortunate because it limits policy understanding of what is ultimately at stake.
In practice, there is significant debate about estimates of the total biomass contained within the ocean subsurface biosphere [44]. This debate can be difficult for an interested observer to understand because it is not entirely clear whether participants are talking about exactly the same thing (e.g. only sediments or sediments and oceanic crust) or using the same measures. In 1998 Whitman estimated that the marine subsurface contains between 27 and 33% of the Earth’s total biomass, a figure much greater than the total estimated biomass in the overlying ocean [45]. Later studies display a trend of reducing this estimate with the most recent studies taking greater account of diverse subsurface environments i.e. those further from hydrothermal activity at mid-ocean ridges or continental margins. Kallmeyer et al. found that total microbial abundance varies between sites by five orders of magnitude and estimated that the marine subsurface biomass is less than 1% of the Earth’s total [36,46]. However, Hinrichs and Inagaki 2012 debate these findings and point out that the “the biomass pool below the seafloor may be ‘only’ as large as the pool above…” [44]. In short, biodiversity in the subsea floor remains highly significant and neglected in debates on marine genetic resources. Greater clarity on this diversity and debates about levels of biodiversity could be achieved through direct engagement with researchers working in this area.
Our ability to directly observe and sample this deep biosphere is limited. However, ocean crust drilling and seafloor observatories increasingly provide a route to insights into the deep biosphere. The key factors in defining habitable zones in the subsurface are temperature, pore space and the availability of energy. A recent study based on ocean drilling shows that microbial life is present not only directly beneath hydrothermal activity, but also at a range of temperatures, crustal ages, fluid compositions and redox conditions [47].
The oceanic crust is 5-6 km thick and is composed of several layers, not including the overlying sediment. In a typical cross section (see Figure 2.5) the topmost layer is comprised of basalt lavas ranging between 500 m and 1 km thick. Below the lavas is a layer of sheeted dikes [48]. These are tabular rock formations, about a metre wide, standing side by side, and are created by periodic bursts of molten rock. The gabbro layer consists of coarse-grained basaltic igneous rock formed from the cooling of molten lava at mid-ocean ridges.
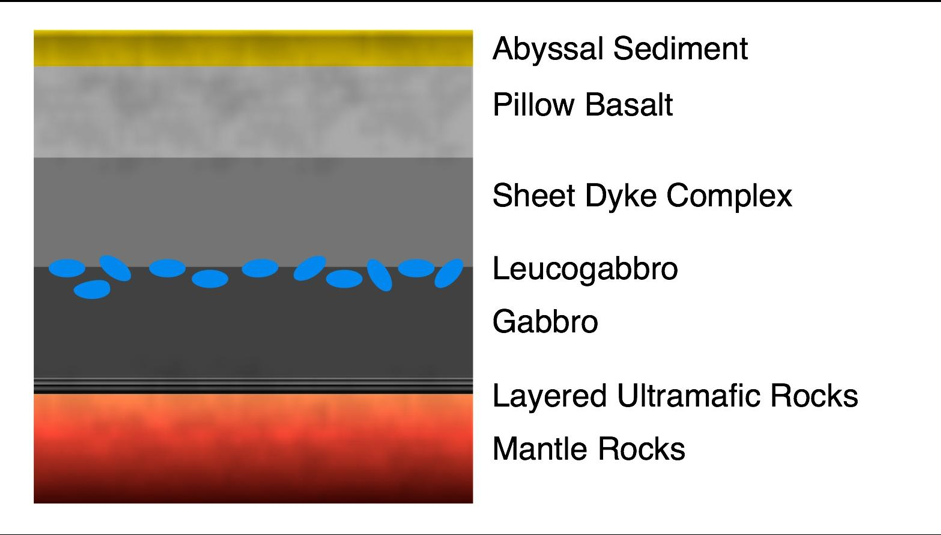
Figure 2.5: Section of typical oceanic crust showing layers as described above (adapted from Heberling 2010)
Inevitably, studies of life in igneous rock have been more difficult than those of life in more accessible seafloor sediments and our understanding of life in the igneous crust is mainly based on samples collected where crustal fluids discharge at the seafloor. Most sampling devices are operated by human-occupied submersibles and remotely operated vehicles (ROVs) although improved sampling devices are allowing more in situ filtration, preservation and isolation of samples. Ocean crust observatories are a key development in this area of research (see below).
While oceanic crust may typically be thought of as a pure solid, in practice igneous crust contains an estimated 2% of the ocean fluid volume and the potentially habitable oceanic crust fluid reservoir is approximately ten times the size of the sedimentary reservoir [47]. Heberling et al. 2010 estimate a biomass of approximately 2 x 10^17 g, 90% of which occurs in the extrusive basalt layer [48].
There have been only few successes in isolating organisms directly from the oceanic crust, mainly due to the difficulty of obtaining uncontaminated samples and the low sample biomass. Orcutt et al., (2011) reported the first results from in situ ocean crust observatories known as CORKs (Circulation Obviation Retrofit Kit) in young (3.5 million years old) basaltic crust in the Juan de Fuca Ridge [35]. The study was based on obtaining 16S ribosomal RNA (rRNA) sequences for comparison with known sequences to identify types of organism [35]. 16S bacterial sequences were prevalent and many were not closely related to previously cultivated microorganisms. Archaeal communities had relatively low diversity and sequences were often grouped closely with known cultivated species. This research revealed that contrary to previous opinion, subsurface microbial populations are dynamic, changing over a relatively short time frame [35]. This suggests the need for time-series observations. As of 2011, there were 34 borehole observatories in basaltic crust - established during ocean drilling expeditions [35,47]. As Edwards et al. 2011 have argued, the future of research in the deep biosphere lies with these deep ocean observatories but requires a new mindset in terms of research timeframes:
Conducting decade-long studies in experimental field microbiology requires fundamental new approaches to the way that microbiologists conduct science; this effectively represents a paradigm shift from, for example, our typical training in the laboratory using well-characterized model isolates for which an experiment can be constructed and conducted over-night. By contrast, microbiological research in the oceanic crust requires long-term planning and demands multidisciplinary approaches and collaborations. This research must approach the scientific endeavor from an Earth–system-science perspective, in which experiments and observations are used to formulate conceptual and numerical models that can inform further iterations of experiments and observations. Such approaches are well established in fields such as geophysics, but are far from commonplace in the microbiological sciences.
[47] at 710)
In our view the question of the extent of biodiversity in the deep biosphere is far from settled. Rather, as the work of Orcutt and Edwards ably demonstrates, a serious rethink of the time-frames and technologies required for investigating life in the deep biosphere is required. Supporting such research will also require a longer-term strategy beyond short-term expeditions and more closely resembles the requirements for the exploration of space than typical terrestrial research. We highlight this here because it indicates the need for long-term government investment and international interdisciplinary collaboration rather than the short-term imperatives that frequently drive commercially oriented research and existing debates on access and benefit sharing.
Seamounts and Knolls
Seamounts are underwater mountains rising above 1000 m but not reaching the ocean surface. Knolls are their smaller counterparts, rising between 500 m–1,000 m. In 2011, 33,452 seamounts and 138,412 knolls had been mapped with total area of habitat being 17.2 million km2 and 59 million km2 respectively [49].
Seamounts are one of the most important marine ecosystems due to their abundance and the diversity of life they sustain. With such wide geographic and bathymetric variety, it is difficult to generalise about benthic communities either within or between seamounts, although it has been generally accepted that they are significant ‘hotspots’ of activity for a diverse range of species. Recent studies suggest that seamounts host communities similar to those in their surrounding environments with little endemism but significantly elevated abundance [50]. However, these observations must be tempered by the realisation that only around 300 seamounts have been studied in sufficient biological detail to consider their specific populations and existing surveys remains biased towards larger organisms [50]. In short, as the scientific literature consistently points out, we remain remarkably ignorant of biodiversity in the deep-sea.
(Source data: Yesson et al. 2011 [49])
The abundance of life at seamounts may be due to upwelling currents formed where they disrupt water flow at the seafloor leading to above-average plankton populations where fish aggregate and attract predators higher up the food chain. In addition, the substrate of seamounts is much harder than the surrounding sedimentary deep-sea floor as they are formed from volcanic rock. This surface supports high populations of suspension feeders, especially those from the phylum Cnidaria that includes corals, sea anemones, sea pens and hydroids [51]. These are long-lived slow-growing invertebrates with low tolerance to physical disturbances [51]. Soft sediments dominate some seamounts, notably those with flat tops known as guyots. Here communities are dominated by deposit feeders such as the polycheates found in other deep-sea sediments.4
Due to large aggregations of fish at seamounts they are under significant threat from over-fishing by commercial fishing trawlers [49]. Over-fishing has had a huge impact on stocks of demersal (bottom feeding) species and trawling equipment has inflicted significant damage on seamount habitats of which only 1.5% fall within Marine Protected Areas (MPAs) [49]. This figure is even lower at 0.7% for knolls [49].
Mineral extraction poses an additional emerging threat to seamount environments. In addition to Seafloor Massive Sulfide (SMS) mining activity proposed at deposits on seamounts with hydrothermal venting (see below), there is interest in mining cobalt-rich crusts on seamounts in the central Pacific Ocean [51,52]. In studying fauna on the central North Pacific Hawaiian Seamount Chain, research concluded that benthos of sites inside cobalt-rich crust areas differs significantly in terms of species composition from that outside the cobalt-rich crust area, with each area having a comparable number of unique taxa and comparable richness [51].
Cold-Water Coral Reefs
Coral ecosystems, although normally associated with shallow, tropical seas, can be found in a wide range of deep-sea locations ranging across continental shelves, seamounts and ridge systems. Deep-sea cold-water corals can be found in small isolated colonies as well as in ancient reefs, sometimes as massive carbonate mounds up to 300 m high [53]. Corals are Cnidarians that often form structures supported by their skeletons, which grow over time to form bank and reef structures. These structures consist of a cap of living coral on top of a framework of dead skeletons. These structures provide habitats for other species that form the communities of the ecosystems [54].
Cold-water corals can be found in waters between 50 m and 1000 m at higher latitudes, but as deep as 4000 m at low latitudes beneath warm waters. Global distribution of cold-water corals is thought to be linked to seawater carbonate chemistry [53]. Despite their importance as habitat creators, the actual number of records available to develop a true understanding of global distribution remains patchy and distribution probabilities are derived from habitat suitability models [55]. Figure 2.7 shows the distribution of known reefs based on the UNEP–WCMC dataset for the Global Distribution of Cold-water Corals (2005) [56,57]. Colonies and reefs are established over long time periods after coral larvae settle on hard substratum where strong underwater currents prevent the deposition of sediments yet enable a supply of food to reach the corals [54].
Coral reefs have enormous longevity with dating of reefs off NW Africa and along the Mid-Atlantic Ridge suggesting that there has been continual coral growth for 50,000 years at some locations [53]. This longevity and the skeletal structure built up by corals enables them to be used as ‘paleo-environmental archives’, revealing past conditions of the oceans.
Murray Roberts identified three principal threats to coral ecosystems: bottom trawling, deep-sea hydrocarbon mining and acidification [53]. There is evidence that trawling has physically damaged and destroyed reefs [53]. Future mining activities will also have potential to physically destroy the reefs and their communities. Acidification of the seas will lead to areas currently occupied by corals becoming inhospitable as their calcification processes are disrupted [53].
Ocean Trenches and Troughs
Oceanic trenches are long, narrow, steep-sided depressions which occur from 7,300 metres to around 11,000 metres below sea level. They typically form in tectonic plate subduction zones and, according to a Delphi study participant, with few exceptions are normally inside the EEZ. Oceanic troughs are characteristically shallower, shorter, narrower, and topographically gentler than oceanic trenches, occurring at a depth range between 2,300 m and 7,440 m although typical maximum depths lie between 4 and 5 km below sea level. Unlike trenches, oceanic troughs probably owe their origins to a wide variety of geologic mechanisms. Deep-sea trenches and troughs can contain a variety of habitats such as abyssal plains, seamounts, hydrothermal vents and cold seeps.
Research in deep-sea trenches is constrained by some of the most extreme conditions the ocean presents. Nevertheless, the attraction of exploring the deepest known part of our oceans has led to a number of expeditions at Challenger Deep in the Mariana Trench, central west Pacific, which, with a maximum known depth of almost 11,000 m, is the deepest known place on Earth. Recent sampling has shown that although the presence of macrofauna is restricted at Challenger Deep, rates of biological consumption of oxygen are high, exceeding rates at a nearby 6,000 m deep site by a factor of two [31]. In addition, “analyses of sediments collected from the two sites consistently reveal higher concentrations of microbial cells at Challenger Deep.” The authors of this study conclude “that the elevated deposition of organic matter at Challenger Deep maintains intensified microbial activity” [31].
Mostly being situated along continental margins, most deep trenches are in close proximity to coastal oceans and transport routes allowing substantial deposition of matter [31]. Ocean currents, landslide and debris flows ensure that sediments and other materials are transported throughout the troughs and canyons, and these processes enable the dispersal of marine organisms. However, it also provides a means for human-made litter to be deposited far from its source. Research at Monterey Canyon has studied the potential impact of pollution over a 22 year period. The authors found that the highest frequency of metal and plastic debris occurred at below 2000 m and that over a third of the observations showed megafauna in close contact with debris [58].
Cold Seeps and Mud Volcanoes
Dr. Charles Paull first discovered cold seeps in the Gulf of Mexico in 1983 [59]. They are now known to exist in all oceans. They are patchily distributed and occur most frequently near ocean margins and along continental margins in areas of high tectonic activity where crustal deformation and compaction drive emissions of methane rich fluid. Topographic features may be depressions (pockmarks) or, equally common, highs such as mounds, mud diapirs and mud volcanoes [59].
At cold seeps, reduced sulfur and methane emerge from the sea floor unheated by volcanic activity. Thiotrophic (sulfur oxidising) and methanotrophic symbiotic bacteria (Euryarchaea) fuel most of the megafauna at these sites which is dominated by tube-worms, mussels and clams with cladorhizid sponges, gastropods and shrimp sometimes abundant [59].
Seep habitats have greater macrofaunal abundance than background sediments, particularly at lower depths. Seep infauna (fauna living in sediment) include organisms which tolerate high sulfide or with behaviours that avoid the toxicity of sulfide. Within the Polychaeta (worms), Dorvilleide are particularly abundant at some sites (the Gulf of Mexico and the Norwegian margin) with Ampharetids dominant at New Zealand and Costa Rica study sites. While these Polychaeta were widespread at all seep habitats, other groups dominated at just one or a few locations. Less sulfidic cold seep habitats tend to exhibit the greatest biological diversity with features such as clam beds [59].
Mud volcanoes are specific cold seep structures where emissions emerge as gas-fluidised mud and the methane may escape as gas bubbles or remain stored as gas hydrates [60]. There are an estimated 100,000 mud volcanoes in the deep-sea [61]. Studies of specific mud volcano systems have considered the positive contribution biodiversity plays in oxidising methane as well as the effects of methane emissions on biodiversity. In a complex system of mud volcanoes in the Mediterranean Sea, it was found that there was lower macro and meiofaunal diversity where there is highest fluid emission, with nematodes being the most abundant taxa at all sites, accounting for 85-94% of taxa, followed by copepods accounting for 5-12% [60]. Types of nematodes vary across different systems of mud volcanoes suggesting that cold seep systems may differ significantly in their biodiversity and indicating the need to protect the contribution of nematodes to marine ecology [60].
Research at the Haakon Mosby mud volcano in the Barents Sea found that methane oxidation was the main source of energy for microbial organisms across the mud volcano’s various habitats and that methanotrophs play an important part in methane removal that is affected by flows of sulfate and oxygen-free fluids [62]. These methanotrophic organisms form part of a wider “benthic filter for methane” signalling their importance in influencing methane entering the wider hydrosphere and atmosphere [63]. Seeps along the global continental margins have been estimated to contribute between 1 and 5% of global methane emissions to the atmosphere [63]. Because of their relevance for oceanic emissions of climate-relevant methane and their biological richness Boetius and Wenzhöfer (2013) argue that cold seeps should be considered key candidates for ecosystem monitoring and protection [63].
Mud volcanoes are also of significant interest for their commercial potential as reserves of gas hydrates. Gas hydrates form when fluids rise from the deep subsurface and crystallise on meeting the cooler temperatures of the surrounding sediments. Based on Milkov’s estimates the approximate total volume of gas hydrate in mud volcanoes is 1010 - 1012 m3 [61]. There is significant interest in exploiting marine gas hydrates for energy, with estimates of its economic value varying widely from 50 times greater than the world’s conventional gas resource to around half of the conventional gas resource (estimates taken from Milkov and Sassen 2002) [64–66]. With the first full production scheduled to start in 2016-18 at the Nankai Trough (Japan) and recovery of marine gas hydrates being economically feasible in a number of other regions, there is major business potential in extracting gas hydrate reserves in the near future [66]. Alongside this economic potential lie the possible negative impacts on the biologically valuable chemosynthetic communities that inhabit these habitats.
Hydrothermal Vents
Hydrothermal vents can reasonably be described as the functional equivalent of the charismatic megafauna that are the icons of international conservation. That is, they receive most of the attention in debates on the deep-sea. In approaching deep-sea biodiversity from the perspective of the water column, the abyssal plain and marine sediment, the oceanic crust, sea mounts, cold seeps and mud volcanoes we have sought to widen the discussion of deep-sea biodiversity and habitats in discussions under UNCLOS.
However, it is undoubtedly the case that the discovery of hydrothermal vents in 1977 provided a new perception of the ocean floor as a dynamic environment with rich benthic communities and radically expanded scientific understanding of the origins and limits for life [42]. Extremophile organisms, thriving at conditions considered to be at the known limits for life in terms of pressure, temperature, pH and chemical composition, have been a major focus of investigation not only in defining these limits but also in the potential for harnessing these properties.
Hydrothermal vents in the deep ocean typically form along the mid-ocean ridges such as the East Pacific Rise and the Mid-Atlantic Ridge. These are locations where two tectonic plates are diverging and new crust is being formed. The geochemistry of vent fluids and deposits varies. Those at fast-spreading ridges such as the East Pacific Rise are dominated by sulfide while those at slow-spreading ridges such as the Mid-Atlantic Ridge are dominated by hydrogen and methane [41]. More unusual are the iron dominated Loihi vents in Hawaii and the carbon dioxide dominated vents of the Okinawa trough [41]. The geochemical composition of vents is dynamic, and the microbial life forms inhabiting these systems are likely to differ not only between vent systems but also within a vent. Differences in chemical composition at spatial scales as small as centimetres and temporal scales of minutes will have an impact on microbial communities and make research more complex, highlighting the need for more long term in situ programmes [41].
Some hydrothermal vents form chimney like structures known as black or white ‘smokers’. A black smoker is found typically in the abyssal and hadal zones. The black smokers typically emit dark particles with high levels of sulfides. Black smokers are formed in fields hundreds of metres wide when superheated sulfide rich water from below the crust comes through the ocean floor (see Figure 2.8). When the heated fluid comes into contact with cold ocean water, many minerals precipitate, forming a black chimney around each vent. The deposited metal sulfides can become massive sulfide ore deposits in time. White smoker vents emit lighter-hued minerals, such as those containing barium, calcium and silicon and tend to have lower temperature plumes.
Many microorganisms at hydrothermal vents are chemoautotrophic, using the oxidation of inorganic molecules or methane as a source of energy. The chemoautotrophic bacteria grow into a thick mat that attracts organisms that Jorgensen describes as the ‘icons of vent life’: tubeworms, molluscs and shrimp [41]. Studies of fauna at geographically distant vents reveal significant heterogeneity. For example, research on the East Scotia Ridge in the Southern Ocean discovered that fauna was dominated by a new type of yeti crab, Kiwa n. sp., stalked barnacles, limpets, peltospiroid gastropods, anemones and a predatory sea star [67]. Taxa common in other vent systems were often entirely absent; indicating that the influences of the Antarctic environment on vent system communities is significant [67].
Some hydrothermal vents have led to the formation of exploitable mineral resources via deposition of Seafloor Massive Sulfide (SMS) deposits that are of significant interest to mineral exploration companies. Seafloor Massive Sulfides are areas of hard substratum with high base metal and sulfide content along with commercially exploitable concentrations of gold and silver [52]. The most concentrated SMS deposits are found at high intensity hydrothermal systems, typically 200–400 degrees centigrade, and there are now 165 known deposits recorded on the InterRidge database [52]. Recent estimates of global SMS deposits suggest that they occur on average every 100 km along the ocean plate boundaries with approximately 900 modern deposits globally (Hannington et al., 2011, cited in Boschen et al., 2013).
In closing this discussion of important deep-sea habitats we provide a table summarising the key features of the habitats discussed above in Figure 2.9. We now turn to analysis of the existing state of knowledge of the impacts of human activity on deep-sea biodiversity and habitats.
Figure 2.9: Summary of the Principal Habitat Types Found in the Deep-Sea.
Type | Habitat | Distribution | Status |
---|---|---|---|
The Water Column | Open waters from the surface to the seafloor. | All open waters are a part of the water column divided into different pelagic zones based on depth. | 50% of biodiversity records are from the continental slopes. The deep pelagic zone (below 200 m) is the most under-represented area in databases. |
The Abyssal Plain | The vast, flat, sediment covered plains between continental margins and mid-ocean ridge systems. | Most of the deep-seafloor is comprised of abyssal plains. They cover approximately 50% of the Earth’s surface. | Understanding of biodiversity limited to relatively few samples due to scale of area and inaccessibility. Location of manganese nodule fields. |
Seafloor and sub-seafloor Sediments | The sediment layer covering much of ocean floor. | Can be found on any of the seafloor habitats and vary in thickness from a few centimetres to 4 km. | Sediments are dominated by microbial life that plays an important role in oxidising methane. |
Oceanic Crust | The rocky crust of the ocean floor beneath sediment layers. | Up to 6 km thick contains several volcanic rock and mineral layers. | Estimates of the microbial biomass vary and our ability to sample is extremely limited. |
Seamounts and Knolls | Seamounts are underwater mountains rising >1000 m from the seafloor (knolls are smaller rising up to 1000 m). | Found across all the oceans and form the longest mountain chains on the planet. | Considered hotspots of biodiversity due to upwelling of nutrient rich water and a diverse range of habitats. Only 350 seamounts (out of 30,000) have been studied. Massive sulfide deposits attracting mining interest. |
Cold Water Corals | Often ancient reefs and colonies of corals forming complex habitats in deep waters. | Found in a wide range of locations including ridge systems, seamounts and continental shelves. | At risk of destruction by bottom trawling and acidification due to their calcification being disrupted. |
Ocean Troughs and Trenches | Trenches are long, deep depressions between 7300 m and 11000 m deep. Troughs are shallower and gentler. | Trenches are found at tectonic subduction zones. Troughs are caused by a variety of geological mechanisms. | The deepest places on Earth. Macrofauna is limited but microfauna can thrive due to organic matter deposits. |
Cold Seeps and Mud Volcanoes | Where reduced sulfur and methane emerge unheated from the seafloor. In mud volcanoes it emerges as fluidised mud. | Found frequently near ocean margins and along continental margins where there is tectonic activity. | Thiotrophic and methanotrophic symbiotic bacteria fuel adapted megafauna. Gas hydrate deposits below mud volcanoes are of commercial interest. |
Hydrothermal Vents | A feature from which super-heated water rich in chemicals from the sub seafloor crust emerges. | Typically found at the mid-ocean ridges where tectonic plates are diverging. | Extremophiles dominate fauna. Black & white smokers deposit minerals, which have potential commercial value. |
2.0.3 Human Impacts on Deep-Sea Habitats
The discussion above has highlighted some of the main potential and actual human impacts on the deep-sea. In the context of the Census of Marine Life, the SYNDEEP project team led by Ramirez-Llodra has provided the most comprehensive overview to date of the main impacts of human activity [68]. Figure 2.10 provides a brief summary of the main findings of this research.
Figure 2.10: Key Threats and Impacts for Marine Habitats [68]
Litter and Waste DisposalMaterials | Methods | Impact |
---|---|---|
Plastics, metals etc. | Accumulate in trenches. Plastics dispersed as micro-particles in the water column. | Consumed by marine organisms. |
Scientific survey waste | Submersible ballast dumped at survey sites. | Pollutes survey sites. |
Large structures | Wrecks and lost shipping containers resting on seafloor. | Can create new hard strata habitats; corrosion and leaking can release pollutants. |
Low level radioactive waste | Historic systematic dumping of barrels of waste in deep ocean. Lost nuclear powered submarines. | Leaking of radionuclides into open waters. |
Persistent organic chemicals | Industrial waste and sewerage carried down into the deep-sea by dense shelf water cascading events and currents. | Chemical contaminants found in high trophic level organisms. |
Discarded fish carcasses | By-catch and other animal parts dumped overboard from fishing vessels. | Provides feed for gulls & seals. Reduces oxygen levels in deeper waters. |
Dead livestock | Animals in transit dumped from vessels when they die. | May provide nutrient hotspots similar to whale falls. |
Storage of CO2 | Proposed storage of CO2 in deep-sea sediments. | Likely to increase acidification of oceans and create toxic plumes. |
Resource Exploitation
Materials | Methods | Impact |
---|---|---|
Fisheries | Technological advances allowing fisheries to harvest from deeper waters. | Damage to habitats by trawls; depletion of cold water (slow breeding) species; stock collapse. |
Mining | Extraction of manganese nodules, mining of cobalt-rich crust and massive sulfide deposits. | Destruction of abyssal plain and seamount habitats; death of organisms in biogeographically isolated sites; pollution from machinery and slurry. |
Oil & Gas | Established industry and potential for gas hydrate extraction. | Potential for spills which can destroy deep-sea communities as plumes cover large areas. |
Bioprospecting | Possible harvesting and sampling of marine life for novel products. | Potential for damage to habitats and marine communities. |
Climate Change
Materials | Methods | Impact |
---|---|---|
Acidification | Lowering pH reduces the ability of water to hold calcium carbonate. | Vital structural corals and other calcifying fauna are unable to survive. |
Temperature Rise | Warmer waters are less able to hold oxygen. | Vertical habitat compression will change ecosystem functions. Productivity falls. Methane hydrate deposits destabilised. |
Invasive Species
Materials | Methods | Impact |
---|---|---|
Species transported to new locations (e.g. in ballast water tanks) | Invasive species outcompete native and endemic species. | Structures of deep-sea communities changed. |
We now turn to a brief discussion of marine protected areas and Ecologically or Biologically Significant Areas (EBSAs).
Marine Protected Areas and Ecologically or Biologically Significant Areas
Marine Protected Areas (MPAs) are zones of the seas and coasts where wildlife is protected from damage and disturbance. MPA is a broad umbrella term for a range of protected areas that serve as the principal vehicles for marine conservation efforts. They are an integral aspect of a country’s environmental policy and are normally managed and monitored by an appropriate government body. Taking the UK as an example, responsibility for the management of MPAs is held by Natural England; Scottish Natural Heritage; Natural Resources Wales and the Northern Ireland Environment Agency. The UK Government has expressed a commitment to establishing a well-managed ecologically coherent network of MPAs in its seas. Natural England defines its role in MPAs in English waters with the following five statements, and this gives a sense of the diverse reasons a country may have for establishing an MPA.
Protect and restore the ecosystems in our seas and around our coasts.
Ensure that the species and habitats found there can thrive and are not threatened or damaged.
Maintain a diverse range of marine life that can be resistant to changes brought about by physical disturbance, pollution and climate change.
Provide areas where the public can enjoy a healthy marine environment learn about marine life and enjoy activities such as diving, photography, exploring rock pools and coastal walking.
Provide natural areas for scientific study.6
MPAs are also established in order to fulfil international obligations, including those in the Convention on Biological Diversity and the EU Marine Strategy Framework Directive. The range of protected areas in UK waters can be seen in Figure 2.11
Figure 2.11: Marine Protected Areas in British waters.
Designation | Type of Protection |
---|---|
Special Area Of Conservation | Protected Marine Habitat or Species of Marine Importance. |
Special Protection Area | Protection of Birds of European Importance. |
Marine Conservation Zones | Protecting nationally important habitats, species and geologies. |
Site of Special Scientific Interest | Most SSSIs are terrestrial but some extend into the marine environment. |
RAMSAR sites | Protected international sites for wetland birds. |
Particularly Sensitive Sea Areas (PSSAs) | An area recognised for its ecological or socio-economic or scientific importance given protection by the International Maritime Organisation. |
At a regional level, a key part of the Convention for the Protection of the Marine Environment of the North-East Atlantic’s (OSPAR) biodiversity strategy for example, is to establish a network of MPAs which is both ecologically coherent and well-managed by 2016. In the period 2005–2012 OSPAR Contracting Parties bordering the North-East Atlantic have selected and nominated sites as components of the OSPAR Network of MPAs. Reflecting that the OPSAR maritime area is composed of areas within and beyond national jurisdiction, MPAs have also been designated in the high seas. Similarly, the Commission for the Conservation of Antarctic Marine Living Resources (CCAMLR) is also establishing MPAs outside of EEZs and continental shelf areas.
Globally, there are at least 4,435 MPAs [69]. There is much guidance available concerning the management of MPAs, with individual governments determining the management and governance of their own MPAs.7 The IUCN provides guidelines for the application of management categories to MPAs for which their definition reads, “A protected area is a clearly defined geographical space, recognised, dedicated and managed, through legal or other effective means, to achieve the long-term conservation of nature with associated ecosystem services and cultural values”8. The governance of MPAs is discussed in the UNEP 2011 report ‘Governing Marine Protected Areas – Getting the Balance Right’ which concludes that there are three perspectives that need to be considered in decision making about governance. The three perspectives are: state regulations, providing a legal framework; community-based approaches to ensure decentralization and local empowerment; and market incentives to ensure alternative livelihoods and that an economic value is attached to biodiversity. The effectiveness of these approaches in managing and governing MPAs is of importance since to date, MPAs in areas under national jurisdiction are the primary areas given biodiversity protection and the knowledge and methods acquired at these locations will be of use in determining approaches to protecting Areas Beyond National Jurisdiction.
Ecologically or Biologically Significant Marine Areas (EBSAs)
Historically, marine conservation initiatives around the world have been focused on coastal areas within EEZs, as seen with the UK example above. Far less attention has been paid to the open waters and deep seas beyond national jurisdiction. To date, the classification and management of deep-sea habitats with the objectives of protecting biodiversity and maintaining resilience has not been adequately enacted. A major step in rectifying this imbalance is the identification of Ecologically or Biologically Significant Areas (EBSAs).
In the UK, the Government supports the principle of designating MPAs in international waters, and specifically supported the call in 2010 by governments of the States party to the Convention on Biological Diversity (CBD) to strive for MPA and other area based mechanisms covering 10% of our oceans by 2020. The UK has therefore made a commitment to the identification of EBSAs, which are areas of the oceans that are recognised as being of greater scientific importance than other areas. EBSAs have been defined by the Conference of the Parties (COP) to the CBD as:
…geographically discrete areas that provide important services to one or more species/populations of an ecosystem as a whole, compared to other surrounding areas or areas of similar ecological characteristics, or otherwise meet the criteria as identified in annex 1 to decision IX/20
(decision IX/20, Annex II)
A set of criteria to identify EBSAs was agreed at the 9th CBD COP in Bonn in 2008 (decision IX/20). The aim of the criteria was to identify the areas of the oceans in need of protection and to provide the scientific guidance required, using the precautionary approach and the ecosystem approach, and to establish a network of representative marine protected areas across the world. Seven key criteria were agreed by the Conference of the Parties for selecting EBSAs as provided in Figure 2.12.
At the 10th Conference of the Parties to the CBD, governments reiterated the central role of the United Nations General Assembly in addressing conservation and sustainable use of biodiversity in marine areas beyond national jurisdiction and the universal character of the United Nations Convention on the Law of the Sea (decision X/29). The COP also promoted collaboration between the CBD and other relevant agencies in the provision of scientific and technical advice to ensure that the best scientific studies were used to identify these priority areas and to work to develop databases to further increase knowledge of these areas (decision X/29).
Figure 2.12: Scientific Criteria Used to Identify a Potential EBSA, adapted from decision IX/20, Annex I.9
Criteria | Definition |
---|---|
Uniqueness or rarity. | Area contains either (i) unique, rare or endemic species, populations or communities, and/or (ii) unique, rare or distinct habitats or ecosystems; and/or (iii) unique or unusual geomorphological or oceanographic features. |
Special importance for life history of species. | Areas that are required for a population to survive and thrive. |
Importance for threatened, endangered or declining species and/or habitats. | Areas containing habitat for the survival and recovery of endangered, threatened, declining species or areas with significant assemblages of such species. |
Vulnerability, fragility, sensitivity, or slow recovery. | Areas that contain a relatively high proportion of sensitive habitats, biotopes or species that are functionally fragile (highly susceptible to degradation or depletion by human activity or by natural events) or with slow recovery. |
Biological productivity. | Areas containing species, populations or communities with comparatively higher natural biological productivity. |
Biological diversity. | Areas containing comparatively higher diversity of ecosystems, habitats, communities or species, or which have higher genetic diversity. |
Naturalness. | Areas with a comparatively higher degree of naturalness as a result of the lack of, or low level of, human-induced disturbance or degradation. |
In 2009 The Global Ocean Biodiversity Initiative (GOBI), a collaboration between intergovernmental organisations, NGOs and academic institutions, was established to provide further technical assistance to states and other regional and global organisations in identifying ecologically significant areas and how they could be effectively managed, developed and monitored to create a network of representative marine protected areas.
The process of identifying an EBSA begins with the selection of a potential site either by the recommendation of experts or by a systematic approach. In 2010 an identification process based on regional workshops was adopted in order to facilitate the description of EBSAs. The summary reports of these regional workshops, once endorsed by the CBD COP, are forwarded to the UN General Assembly, which is recognized as being the competent arena to discuss any future policy implications with respect to EBSAs identified in Areas Beyond National Jurisdiction.
EBSAs are a scientific tool which provide an important starting point for future research and monitoring. Thus far 63 areas have been described as meeting the EBSA criteria with additional EBSAs proposed in areas such as the NE Atlantic. Figure 2.13 illustrates these areas based on information provided by the EBSA website of the Secretariat of the CBD and Defra in the UK10.
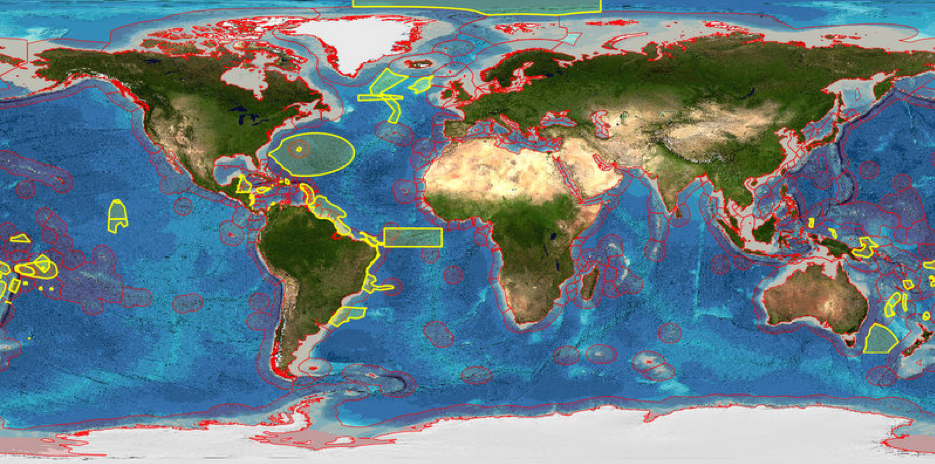
Figure 2.13: Global distribution of EBSAs (yellow) and EEZs (red)
Other models for EBSAs have been developed outside of the CBD. The most notable are those of Fisheries and Oceans Canada (DFO) and the FAO whose model is known as Vulnerable Marine Ecosystems and focuses on the impact of deep-sea fisheries. In considering whether the three systems can be reconciled, Gregr et al., (2012) discuss the complexity of classification of marine environments and the lack of a single universal methodology [70]. Gregr et al., (2012) examined 16 classification methods (using physical, biological and ecological approaches) to determine a new best approach. They argue that an integrated methodology, combining data of both biological origin and physical features, can be created to be more successful in encompassing biologically sensitive areas and important marine features and which is practicable for use in a repeatable manner. More than anything their research demonstrates that the process of classifying areas of the ocean based on the EBSA criteria is both complex and still developing.
In a similar vein, Gerald Taranto et al., (2012) seek to develop a methodology for the identification of individual seamounts for which an EBSA designation would be appropriate [71]. They argue that many deep-sea habitats are dynamic, and in the case of pelagic habitats not geographically fixed. Seamounts, having such an important role for pelagic species, would therefore be excellent candidates for special protection. In a series of studies of named seamounts, EBSA criteria were applied looking at features such as vents and their communities, cold-water coral reefs and threatened visiting pelagic fauna as indicators. The paper concludes that the identification and protection of important seamounts would achieve the conservation goals of the CBD when combined with spatial analysis of human activities such as mining and fishing.
As this brief discussion makes clear, EBSAs are an emerging instrument for the conservation and sustainable use of marine biodiversity in Areas Beyond National Jurisdiction. One question that we will contribute to addressing in the course of this report is the extent to which existing EBSA coverage correlates with known areas of scientific research and patent activity.
Marine Ecosystem Services
Ocean ecosystems are a complex web of habitats and organisms. The communities of organisms are often highly diverse and provide a wide range of ecosystem goods, services and benefits to human society. Collectively these benefits are known as ecosystem services and they provide an inclusive means of engaging economically, culturally, and scientifically with the oceans [72].
The following is taken from the UK National Ecosystem Assessment Technical Report chapter on Marine ecosystem services [72]. Ecosystem Services can be grouped into four main areas: provisioning, regulation, cultural and supporting, as illustrated in figure 2.14 below.
A wide range of provisioning, regulating and cultural services are provided by the deep oceans. Nutrient recycling by deep-sea fauna provides the necessary foods to fuel surface productivity and thereby maintain fisheries, and microbial metabolic activity is responsible for breaking down toxic compounds, which find their way into the oceans from human activities.
Carbon from the atmosphere is transported by biological processes into the deep-ocean water where it can remain for very long periods of time, compensating for the immediate impact of anthropogenic carbon release. Microbial oxidation of methane prevents much of this even more potent greenhouse element from entering the atmosphere. This indicates the capacity of the oceans to provide a measure of security in the face of climate change.
The deep-sea contains a number of resources that may be extracted or harvested for the benefit of society. At present this is dominated by fish stocks, but in the future there is likely to be a diverse range of products emerging from the oceans’ depths if the potential for bioprospecting activity becomes apparent and achievable. Large amounts of minerals, metals and other elements upon which modern technologies are dependant can be found in the deep seas on seamounts, at hydrothermal vent systems and on the abyssal plains.
Finally, there are the cultural, often non-quantifiable, aspects of the seas and our relationship with them. Society benefits from the mystery and the history of its struggle with the seas. It is the largest environment on the planet and yet one where we cannot go without danger and the aid of technology. It contains life forms which are all but alien to us and reminds us how little we truly know of our home planet.
Figure 2.14: Marine Ecosystem Services [55]
Provisioning | Regulating | Cultural | Supporting |
---|---|---|---|
Food: finfish; shellfish; seaweed; fish oil and fishmeal. Bait for sea angling Aquaculture: farming, with salmon and shellfish being most important to the UK and seaweed being a growing area of aquaculture. Blue biotechnology: pharmaceuticals and biomedical research tools; biocatalysts; fertiliser (seaweed); foulants and anti-foulants; nutritional supplements; personal care products. Energy: Wave and tidal power. Biofuels from macro and micro algae. |
Climate regulation: regulation of biogases such as carbon, organic halides, ozone, oxygen, dimethyl sulfide. Flood, storm and coastal protection: species bind and stabilise sediments and create natural sea defences to dampen the effects of surges, storms and floods (e.g. biogenic reefs, seagrass beds, mudflats and saltmarshes). Pollution control: waste breakdown and detoxification of human and agricultural waste; chemical and industrial waste (including nanoparticles, pharmaceuticals, nitrogen and phosphorus); oil (natural seepage and routine release from shipping). |
Tourism Education Recreation Well-being Heritage Philosophical and inspirational Wild species (including ‘flagship species’) |
Nutrient recycling: Within seabed sediments, water columns; between trophic levels and in the course of bacterial breakdown of detritus. Biologically mediated habitat: Organisms provide living habitats and/or refuge for other organisms through their normal growth, examples in UK being maerl ground (calcified red seaweed), mussel patches and cold water coral reefs. |
2.0.4 Conclusions
The purpose of this chapter has been to introduce the reader to the diversity of marine habitats in Areas Beyond National Jurisdiction drawing on the latest literature on biodiversity and threats to biodiversity. In the process we have introduced instruments such as Marine Protected Areas and new developments such as Ecologically or Biologically Significant Areas (EBSAs) as well as the application of ecosystem services to the valuation of services provided by the marine environment.
In presenting this overview, our purpose has also been to highlight that, in our view, existing debates on access and benefit-sharing in Areas Beyond National Jurisdiction have been too narrowly focused on charismatic locations such as hydrothermal vents. We do not raise this point because hydrothermal vents are lacking in importance. Rather, a broader understanding is required of the scale of marine habitats, the diversity of organisms within those habitats, and the important – if at times neglected – research that is taking place in the middle of the water column, involving cold water corals or exploring the deep biosphere in the oceanic crust. There is life in all of these habitats and any proposed implementing agreement within the framework of UNCLOS will benefit from increasing attention to these diverse locations.
A second and striking feature of this overview is the fundamental problem of human ignorance of the largest environment on this planet. The Census of Marine Life at once expanded our knowledge of the marine environment and at the same time emphasised the profundity of human ignorance of an environment that is already threatened by human intervention. In the context of debates on a possible new agreement within the framework of UNCLOS involving access and benefit-sharing for marine genetic resources this raises the simple question of what should such an agreement be for? Our answer is simple. Any implementing agreement within the framework of UNCLOS on access to genetic resources and benefit-sharing should be directed to advancing human knowledge and understanding of biodiversity in the deep-sea and no other purpose. Improving human understanding of this environment will involve significant investments in research, international research cooperation and a research programme with a time scale measureable in decades.
We now turn to analysis of trends in research on marine genetic resources involving the deep-sea.
Areas Beyond National Jurisdiction as defined by UNCLOS include the water column (the High Seas) and the seabed (the Area).↩
Status of the United Nations Convention on the Law of the Sea, of the Agreement relating to the implementation of Part XI of the Convention and of the Agreement for the implementation of the provisions of the Convention relating to the conservation and management of straddling fish stocks and highly migratory fish stocks. Table recapitulating the status of the Convention and of the related Agreements, as at 10 January 2014. http://www.un.org/depts/los/reference_files/status2010.pdf. Accessed 06/05/2014.↩
http://www.ngo.grida.no/wwfneap/Projects/Reports/Offshore.pdf p17-18 Accessed 30/07/2014↩
Adapted from NOAA/OER 2002 http://oceanexplorer.noaa.gov/explorations/02fire/background/vent_chem/media/chemistry.html Accessed 30/07/2014↩
http://www.naturalengland.gov.uk/ourwork/marine/mpa/default.aspx Accessed 30/04/2014↩
Governing Marine Protected Areas – Getting the Balance Right, Technical Report UNEP. Accessed 26/05/2014 via http://www.mpag.info/governing-mpas-final-technical-report-web-res.pdf↩
Guidelines for Applying the IUCN Protected Area Management Categories to Marine Protected Areas – IUCN Switzerland 2012. Accessed 26/05/2014 via http://www.iucn.org/about/work/programmes/gpap_home/gpap_capacity2/gpap_bpg/?11131/Guidelines-for-Applying-the-IUCN-Protected-Area-Management-Categories-to-Marine-Protected-Areas↩
Azores scientific Criteria and Guidance or identifying ecologically or biologically significant marine areas and designing representative networks of marine protected areas in open ocean waters and deep sea habitats – IUCN 2009 Accessed 09/05/2014 via http://www.iucn.org/about/work/programmes/marine/marine_our_work/marine_mpas/mpa_publications.cfm?uNewsID=4374↩
Data sources: http://www.cbd.int/ebsa/. Accessed 30/07/2014. Shape files were downloaded from the CBD EBSA website and complemented by shapefiles for proposed European EBSAs provided by DEFRA.↩