1 Chapter one: General Introduction
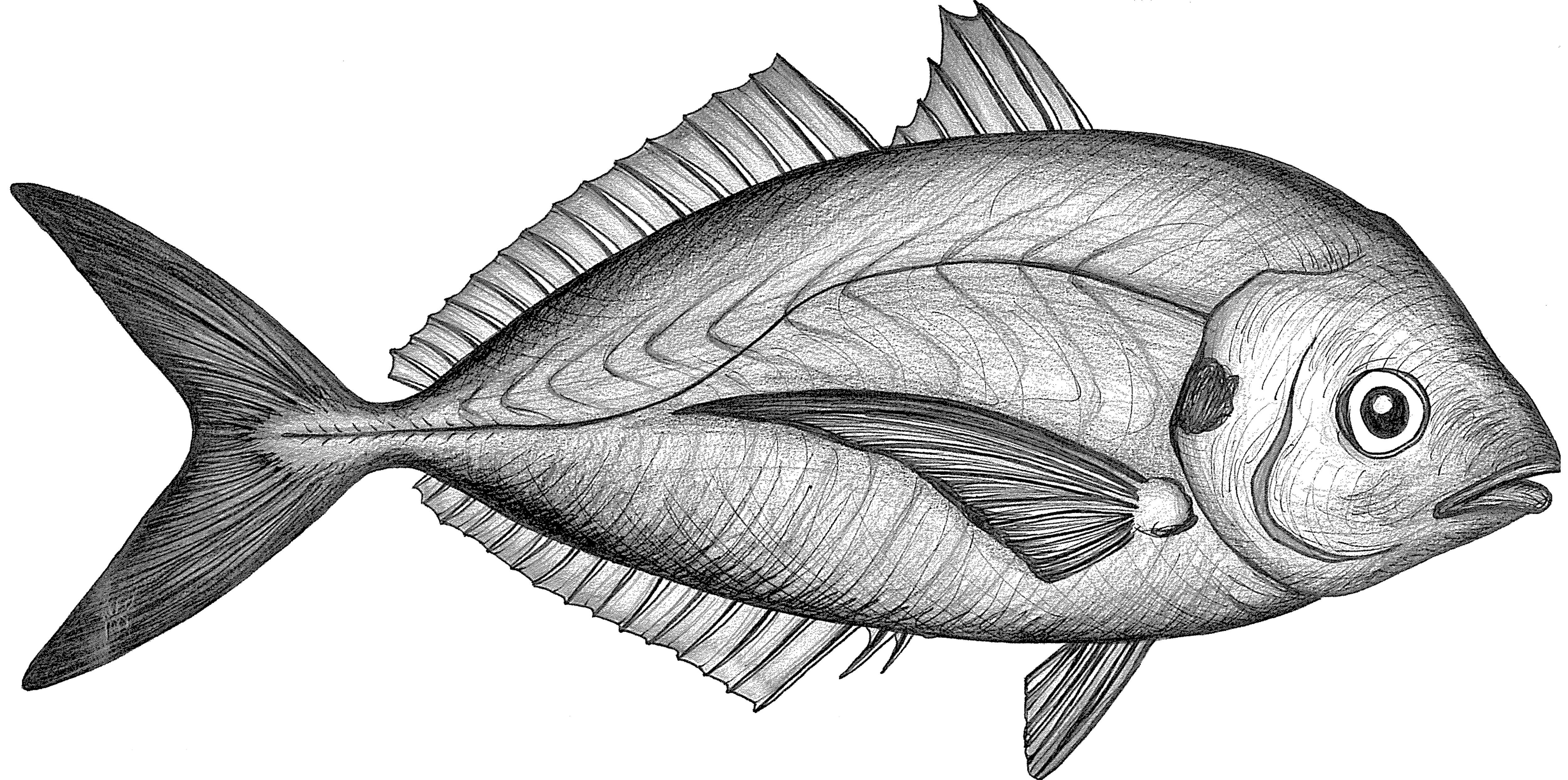
Figure 1.1: Trevally Pseudocaranx georgianus illustration by Leah Kemp.
1.1 Pseudocaranx georgianus
The Carangidae family is a large and diverse family of teleost fish which are globally important for commercial and recreational fishing (Froese and Pauly 2019). The family includes 30 genera of largely marine species such as trevallies, jacks, amberjacks, pompanos, scads, kingfish, pilotfish and queenfishes (Jaafar et al. 2012). The genus Pseudocaranx (Bleeker, 1863) includes the five species of trevallies (Dyer and Westneat 2010; Smith-Vaniz and Jelks 2006) that occupy an extensive range in temperate regions (Smith-Vaniz and Jelks 2006). The greatest number of described Pseudocaranx species occur in Australasia, where four of the five known species occur (Smith-Vaniz and Jelks 2006).
In New Zealand, the single species, Pseudocaranx georgianus (Cuvier, 1833) is known to occur (which also ranges into southern coastal Australian waters) and is distinguished from a similar species by meristic characters (fifteen caudal vertebrae) and morphological features including a blunt snout, a pronounced hump on the forehead and a large and diffuse opercular spot (Smith-Vaniz and Jelks 2006). This species is more commonly known as silver trevally, or by the Māori name Araara. P. georgianus is a commercially important fishery in Australasia (Langley 2004). In New Zealand, over 4000 tonnes are allocated annually for commercial fishing (Fisheries Science Group 2018). The species is also considered to be of high recreational and customary significance with 100 tonnes and one tonne annually allocated for recreational and customary use, respectively (Fisheries Science Group 2018). The importance of inshore fin fish such as P. georgianus are reflected in the National Fisheries Plan which states that “They contribute to our cultural and social traditions, to our nation’s economy and to our sense of overall well-being”.
P. georgianus is both a pelagic and demersal species (Fisheries Science Group 2018) occurring to a depth of 150 meters (James 1976). Juvenile P. georgianus have a typical embryonic development for fish (James 1978) and occupy sheltered inshore areas for up to two years until they enter a demersal phase (Fisheries Science Group 2018). Juvenile P. georgianus experience a moderate growth rate in the first few years of life, although the growth rate drops significantly thereafter (James 1978). Adults live to beyond 40 years and commonly reach 4.5 kilograms and a fork length of 60 centimeters but are known to reach 6-8 kilograms (Fisheries Science Group 2018). Female fecundity is low until females reach about 40 centimeters fork length (Fisheries Science Group 2018). It has been reported that the growth rate of P. georgianus in New Zealand is faster and the maturation age later than for P. georgianus from Australia (Department of Agriculture and Water Resources 2018).
1.1.1 Distribution
Adult P. georgianus are known to occur in inshore and offshore locations in the North and South Island of New Zealand (see Figure 1.2) as well as the southern half of Australia from New South Wales to Perth (Smith-Vaniz and Jelks 2006). Based on data compiled by the National Institute of Water and Atmospheric Research (NIWA), the greatest density of fish occurs in coastal regions (National Institute of Water and Atmospheric Research 2019). In the North Island, these high-density regions occur off Ninety Mile beach, off the east coast of North Cape, the Bay of Plenty, Gisborne, South Taranaki Bight and along most of the east coast of the North Island. In the South Island, a higher density of P. georgianus is found in Tasman Bay off Nelson and northern Karamea Bight (see Figure 1.2).
The juvenile distribution of P. georgianus in New Zealand is limited to coastal regions, predominantly around the North Island but reaching as far south as Bruce bay on the West Coast of the South Island and Kaikoura on the East Coast. The spawning distribution of P. georgianus is even further constrained to inshore regions of only the North Island (see Figure 1.2). There is limited information on P. georgianus spawning regions in the literature, although a putative P. georgianus spawning area has been reported in the 0-25m stratum in Urenui Bay extending north of Tirua point by a study that investigated snapper distribution and growth in the North Taranaki Bight (Horn 1986). In this study, a large number of juvenile P. georgianus were caught in two bottom trawl surveys of the area. Since then, juvenile P. georgianus have been reported at numerous nursery-like locations around northern New Zealand (Francis et al. 2005) and eggs have been observed along the southern Queensland coast (Neira et al. 2015).
There is some variation in the range and distribution of high density regions of P. georgianus associated with season (see Figure 1.3). The high-density region of P. georgianus in the Bay of Plenty occurs in all seasons except for summer. In spring, a second region appears off Whakatane in the eastern most region of the Bay of Plenty. In Autumn, the range of the high-density region in the South Taranaki Bight increases and extends further south. The high-density region in the northern parts of Karamea Bight occurs predominantly in summer and over a smaller range in winter but is not present at all in spring and autumn. In summer, the high-density region in the North Taranaki Bight joins with the largest ranging high-density region along the east coast of the North Island over the North Cape and ranging into Great Exhibition Bay in East Northland. Otherwise these regions are separated in spring, autumn and winter. Seasonal changes in the distribution of juvenile and spawning P. georgianus in New Zealand has not been reported in the literature.
1.1.2 Movement and migration
An important component of population structure is movement and migration of individuals between populations (Laikre, Palm, and Ryman 2005). There is limited information on the movements of New Zealand P. georgianus, however field research typically indicates that the species has surprisingly low levels of movement given the migratory nature of Carangidae species (James 1980). A tagging experiment was undertaken on trawl caught P. georgianus in several locations in the Bay of Plenty and Hauraki Gulf. The 130 tagged fish that were recovered over a five year period indicated that P. georgianus in this region had limited movement, with 88% moving less than 55 kilometers from their release sites (James 1980). The greatest distance observed was 246 kilometers from Great Barrier Island to Whakatane (James 1980). Additionally, a single P. georgianus larval fish swam 82 kilometers in a study that forced larval reef fish to swim in a flume chamber without food or rest (Dudley, Tolimieri, and Montgomery 2000). The literature has not described the levels of P. georgianus movement in other regions of New Zealand.
Recently, a tag-recapture study was undertaken on a substantial number of P. georgianus (over 6300) from 1007 kilometers of the east coast of Australia and over fourteen years (Fowler, Chick, and Stewart 2018). The median movement from the release location to the recapture location was five kilometers and 88.5% of the individuals were recaptured within 50 kilometers of their release location with a time at liberty between one and 427 days. Several individuals were recaptured at their release location. The largest movement recorded was 508 kilometers. Importantly, these tag-recapture studies will likely not capture the extent of fish movements, since it only measures their location at two points in time and is unable to capture non-linear, back-tracing or short term movements.
Acoustic telemetry has been used to investigate the movements of twelve P. georgianus in Western Australia for up to one year. This study found that the home ranges of P. georgianus in Jurien Bay in Western Australia were varied in a diurnal manner being larger during the day (0.49 to 1.29 squared kilometers) and smaller during the night (0.37 to 1.61 squared kilometers) (Fairclough et al. 2011). The home ranges during the day and night were typically at 1-8 meters depth in reef and seagrass habitats, and occasionally sand or patchy seagrass habitats. One fish moved out of the detectable range which is estimated to be able to detect tags 282 kilometers away.
Further research is available on the movement patterns of close relatives of P. georgianus. A long-term monitoring study investigating the habitat usage of P. dentex in the Azores Islands in the Northern Atlantic Ocean captured movement patterns over three time scales (Afonso et al. 2009). Using active and passive acoustic tracking and tag-release capture methods, they observed differences in inshore and offshore fish. For three inshore fish, extensive daily movement was observed (between four and seven kilometers). Daily movement was more limited for three offshore fish (400 meters). Over seasonal time scales, inshore fish moved in a pattern similar to their daily movement, whereas offshore fish showed a strong seasonal migration to inshore or other offshore habitats associated with the summer spawning season. Tag-recapture of 58 fish showed long-term movements from the release locations between 100 meters and 52 kilometers. One individual swam at least 30 kilometers in 48 hours.
Carangidae species typically have a migratory nature (James 1980) and research on P. georgianus and other Pseudocaranx species suggests that adult and juvenile P. georgianus have a strong swimming ability. Despite their apparent capacity for long distance movements, limited movement is often observed with infrequent long distance movements. This may be a result of both an underestimation of P. georgianus movements in tag-recapture studies as well habitat preferences associated with life stage and season. Information on the migration and movement patterns of P. georgianus provide an indication of their potential for long distance migrations and what level of genetic structure of P. georgianus populations is possible. However, it does not indicate whether long distance migrations do occur or whether the migrating individuals reproduce and contribute genetically to resident populations.
1.1.3 Habitat preferences and reproductive behaviour
It appears that factors such as ocean currents, habitat preferences and reproductive behaviour may have an important role in the distribution of adult, juvenile and spawning P. georgianus. P. georgianus appear to exhibit seasonal preferences in habitat use. Adult P. georgianus in New Zealand will move between pelagic and demersal habitats (Fisheries Science Group 2018). They are thought to be batch spawners laying small batches of eggs over several weeks or months over the summer spawning season (Fisheries Science Group 2018) as is the case with P. dentex (Afonso et al. 2009). P. georgianus have been reported by fishermen to move inshore in spring and offshore in summer, although it is unclear if this observation is due to seasonal differences in fishing patterns (Fisheries Science Group 2018). These observations are supported by Afonso et al. (2008) and Afonso et al. (2009) which observed inshore and offshore populations of P. dentex undertake longer range migrations during the spawning season, aggregating around the summits of offshore reefs. Otherwise, these populations are thought to be segregated for most of the year (Afonso et al. (2008), Afonso et al. (2009)). Within a spawning season, a single inshore site was visited by most P. dentex individuals tracked in the study (Afonso et al. 2009). This batch spawning and seasonal migration behaviour is thought to increase mating opportunities for P. dentex (Afonso et al. 2009) and could do the same for P. georgianus.
Pseudocaranx species also exhibit habitat preferences. Juvenile P. georgianus are often found inshore in shallow estuaries and harbours in New Zealand (Fisheries Science Group 2018) and Western Australia (Farmer et al. 2005). A tendency for schools of juvenile P. dentex to use sheltered inshore habitats is thought to be a result of feeding preferences and protection from predators (Afonso et al. 2008). This behavioural tendency to seek refuge was highlighted by a study by Castro, Santiago, and Hernandez-Garcia (1999) observing P. dentex behaviour using Fish Aggregation Devices in the Canary Islands (central-east Atlantic). Additionally, modelling of presence-absence data by Brooks (2019) indicates that baythemetry is an important determinant of P. georgianus occurrence in New Zealand.
The geographic distribution of P. dentex larvae off the coast of Brazil was suggested to be related to factors such as water movements and food availability (Campos, Castro, and Bonecker 2010). Furthermore, juveniles and sub-adults of P. chilensis were possibly transported by currents as larvae to a region over 800 kilometers from where they were previously known to occur (observed on Chanarel Island off the coast of Chile, known to occur on Desvanturadas and Juan Fernandez Islands) (Bearez and Villaroel 2018).
Genetic connectivity of individuals, populations or species can result from either common ancestry or an ongoing reproductive connection of populations. Therefore, to fully understand the taxonomy, population genetic structure and demographic history of P. georgianus and understand their relevance to P. georgianus biology and fisheries management, the analyses undertaken in this thesis will need to interpreted in the light of P. georgianus movement and migration habits, reproductive behaviour and habitat.
1.1.4 Sub-stocks and cryptic species
There is evidence to suggest sub-stocks of P. georgianus and cryptic Pseudocaranx species are occurring within the P. georgianus fisheries in New Zealand. Time-series analysis of catch at-age data suggest that fish from East Northland to Hauraki Gulf and the Bay of Plenty represent sub-stocks of P. georgianus (Fisheries Science Group 2018). Modelling of stock abundance (Fisheries Science Group 2018) and observations in a tag-recapture study (James and Stephenson 1974) suggest that fish from TRE2 and the Bay of Plenty (TRE1) are the same biological stock. Lastly, spatial heterogeneity of age composition and trends in catch per unit effort (CPUE) data suggest three sub-stocks are present within TRE7 on the west coast of New Zealand’s North Island: Ninety Mile Beach, Tauroa Point to North Taranaki Bight and South Taranaki Bight (Fisheries Science Group 2018).
The taxonomy of the Pseudocaranx genus has been historically contentious, largely as a result of their similar morphologies and an underestimation of gene flow between geographically isolated populations (see James and Stephenson (1974) and Smith-Vaniz and Jelks (2006)). In New Zealand, morphological analyses by James and Stephenson (1974) and Smith-Vaniz and Jelks (2006) suggest a species distinct from P. georgianus exists in the Kermadec (Raoul) Island and the North Cape of New Zealand, however which species these represent remained inconclusive. Currently, P. wrighti is only known to occur in the southern half of Australia (Smith-Vaniz and Jelks (2006)). Importantly, mitochondrial DNA studies in Japan have found that morphologically similar P. dentex are genetically distinct (see Masuda et al. (1995) and Yamaoka1992). Furthermore, Pseudocaranx species in Australia often have overlapping intra-species variation in taxonomically distinguishing morphological features (see Froese and Pauly (2019), Smith-Vaniz and Jelks (2006)).
Figure 1.2: Annual distribution of P. georgianus in New Zealand by life stage as indicated in green.
Figure 1.3: Seasonal distribution of P. georgianus in New Zealand as indicated in green.
1.2 P. georgianus commercial fisheries
A rights-based, single species Quota Management System (QMS) was implemented in 1986 to address the legally binding goal to sustainably utilise New Zealand’s fisheries (Cryer, Mace, and Sullivan 2016). Under this system, a total allowable catch (TAC) is allocated for species stocks defined by Quota Management Areas (QMAs) (Cryer, Mace, and Sullivan 2016). Much of the TAC is allocated to commercial fishing as the total allowable commercial catch (TACC) with proportions also allocated to customary, recreational use and other fishing-related mortality (Cryer, Mace, and Sullivan 2016). P. georgianus was introduced into the QMS in 1986 and is managed under five quota management areas; TRE1, TRE2, TRE3, TRE7 and TRE10 (Fisheries Science Group 2018) (see QMA boundaries in Figure 1.4).
The total allowable catch (TACC) of P. georgianus varies by QMA, with the current allowances being 1507 tonnes for TRE1, 241 tonnes for TRE2, 22 tonnes for TRE3, 2153 tonnes for TRE7 and 10 tonnes for TRE10. Fishing intensity is highest in TRE7 and TRE1 as shown in Figure 1.4. Landings in TRE1 experienced a general decline after 1986 when TACC limits were implemented. However, since 2014, fishing intensity in TRE1 have nearly reached levels experienced between 1983 and 1986. Fishing in TRE1 has not exceeded the TACC since its introduction. Fishing intensity in TRE2 is comparatively low and has been consistent for the last 30 years. However, landings in TRE2 have exceeded the TACC in 18 of the last 29 years, exceeding the yearly TACC by up to 127 tonnes. Landings in TRE3 are typically well below the TACC and often a yearly catch will be less than 1 tonne. The most intensive fishing occurs in TRE7 along the east coast of New Zealand’s North Island. Fishing is often under the TACC, although it has been marginally exceeded in 3 of the last 29 years. Often no P. georgianus are taken from TRE10 and a small amount has been taken for only two of the past 29 years. Over the last 31 years, 84.80% of the TACC for all QMA’s combined have been fished (see Table 1.5).
P. georgianus is primarily caught along the northern coasts of the North Island, but fish are also caught as far south as the north of the South Island (Fisheries Science Group 2018). Trawl is the primary fishing method, although purse seine has increased to substantial quantities since the 1970’s particularly in the Bay of Plenty. A modest amount of P. georgianus is also taken by set net (Fisheries Science Group 2018).
P. georgianus is also an important commercial fishery in Australia and is fished within the Commonwealth Trawl and Scalefish Hook Sector on the south-eastern Australia (Australian Government Department of Fisheries 2019a) and the Norfolk Island Fishery in the Norfolk Islands (Australian Government Department of Fisheries 2019b). Fishing intensity was historically high in the Commonwealth Trawl and Scalefish Hook Sector, reaching nearly 1600 tonnes in 1990, but fishing intensity has showed a steady overall decrease to just below 55 tonnes in 2017-18 (Department of Agriculture and Water Resources 2018). Commercial fishing is not permitted within the Norfolk Island Fishery and landings from charter and recreational fishing are comparatively low (Australian Fisheries Management Authority (2010); Australian Government Department of Fisheries (2019b)).
Management of New Zealand fisheries under the Quota Management system assumes that Quota Management Areas (QMA’s) represent single biological stocks of a single species. Therefore, it is imperative to identify any sub-stocks or cryptic species currently included in P. georgianus fisheries to ensure the sustainable management of this Taonga. Currently, limited data is available to validate or reject claims of P. georgianus sub-structure and cryptic Pseudocaranx species in New Zealand waters. This highlights the need for genetic analysis of Pseudocaranx species in Australasia to resolve morphologically inconclusive species occurring in New Zealand P. georgianus fisheries.
1.2.1 Stock status
There is currently no accepted stock assessment of two of the three QMA’s undergoing heavy commercial fishing effort (TRE1 and TRE2) but stock assessment for these areas are underway or planned as further data is collected (Fisheries Science Group 2018). For the most commercially important fishery, TRE7, a stock assessment has been undertaken, but is based only on the core of the fishery from Tauroa point to North Taranaki Bight. Modelling in this stock assessment estimated that female median spawning biomass has declined by roughly half since the 1950’s from over 20,000 metric tonnes to around 10,000 metric tonnes (Fisheries Science Group 2018). This decrease is at times consistent with the rate of increase in the annual catch (Fisheries Science Group 2018). Spawning biomass was comparatively stable during the 1990’s and 2000’s at roughly half the level observed in the 1950’s, but with a slight recovery observed from 2010 (Fisheries Science Group 2018). It is unknown how much P. georgianus is illegally caught or the impact of other sources of mortality such as the degradation of juvenile habitats (Fisheries Science Group 2018). Although the New Zealand P. georgianus fisheries have and are currently undergoing heavy commercial fishing pressure, the fisheries are considered stable (Fisheries Science Group 2018) although further data is required from a broader region within TRE7 and stock assessments are required for TRE1 and TRE3 in order to fully understand the status of New Zealand P. georgianus fisheries.
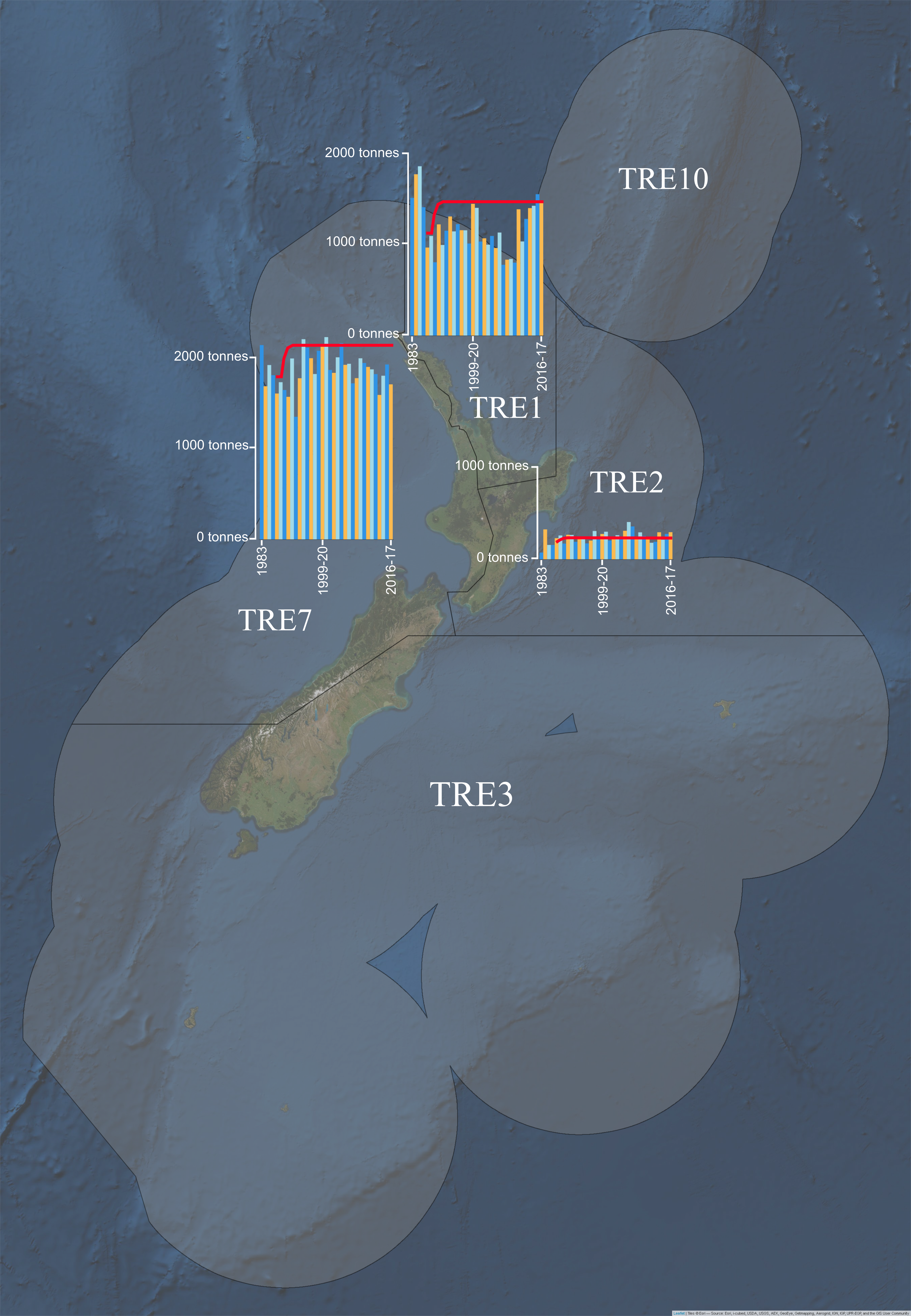
Figure 1.4: P. georgianus Quota Management Areas and yearly fishing effort for the three major commercially fished Quota Management Areas. The total allowable commercial catch (TACC) is indicated in red.
Figure 1.5: Historical fishing effort by Quota Management Area (QMA).
1.3 Molecular genetics
Genetic studies based on mitochondrial DNA have been an important component of population genetic research for several decades (Ballard and Whitlock 2004). Mitochondrial markers in genetic research provides distinct biological and practical advantages, causing such markers to be widely used in phylogenetics and population genetics from the 1990’s (Ballard and Whitlock 2004). One of the primary reasons for this interest is that mitochondria do not undergo recombination because of its maternal mode of inheritance (Avise et al. 1987). This provides a genetic sequence which theoretically has an accumulation of mutations as a result of genetic drift without the contribution of mutations from recombination. For phylogenetics and population genetics, this means that mitochondrial markers are considered largely neutral (Avise et al. 1987). However in recent years it has been recognised that natural selection can have some influence on mitochondrial DNA markers (Ballard and Whitlock 2004).
The mitogenome also has a high mutation rate compared to nuclear markers, particularly the control region (Avise et al. 1987). This has allowed intraspecific analyses at the micro-evolutionary scale (Avise et al. 1987). Mitochondrial DNA markers are also easy to amplify through polymerase chain reaction (Ballard and Whitlock 2004) making mitochondrial DNA studies more straightforward and cost effective than genomic studies. Overall, mitochondrial DNA markers present an opportunity to undertake a low risk ‘first look’ at a species of interest that has undergone little or no genetic research.
Today, we are transitioning into the genomics era, as producing whole genome data is becoming more affordable and more sophisticated statistical and computational software is developed (Casillas and Barbadilla 2017). As we stand on the precipice of the genomics era, time and money constraints still encourage more traditional mitochondrial DNA studies, particularly as a first genetic investigation into a species for which little genetic research has been undertaken.
1.3.1 Phylogenetics
One of the most powerful statistical advances in population genetics over the last few decades was the introduction of the stochastic process model referred to as ‘the coalescent’ (Kingman 2000). This has enabled researchers to account for randomness inherent in evolution (Rosenberg and Nordborg 2002) and a produced a ‘tree thinking’ that has transformed many branches of biology (Yang and Rannala 2012).
These theoretical developments combined with the increased availability of genetic data has progressed the field of phylogenetics from a field based on systematics and taxonomy to a field dominated by molecular genetics (Yang and Rannala 2012). Constructing phylogenetic trees from genetic polymorphisms allow us to model the evolutionary history of organisms (Rosenberg and Nordborg 2002) including the persistence of genetic lineages through time as well as species relationships and common ancestry (Yang and Rannala 2012).
There are several methods of phylogenetic inference, presenting advantages and disadvantages which are discussed in more detail elsewhere (see Yang and Rannala (2012)). However, maximum likelihood and Bayesian inference methods provide advantages over distance matrix and maximum parsimony methods since they can model sequence evolution more realistically (Yang and Rannala 2012) when appropriate model priors are used (Huelsenbeck and Rannala 2004).
1.3.2 Population genetics
The field of molecular population genetics arose in the 1966, born from a theoretical platform of population level concepts of evolution such as Mendelian inheritance concepts of changing allele frequencies in populations and the Darwinian concept that biological evolution of genetically distinct species arise from genetic variation in populations (Casillas and Barbadilla 2017). For some time, there existed an extensive theoretical foundation for molecular population genetics, however recent technological advances have permitted the field of molecular population genetics to flourish and diversify (Casillas and Barbadilla 2017). Molecular genetics now has a varied range of applications in ecology, evolution and conservation (Kress et al. 2015).
1.3.2.1 Population structure
There are three main types of genetic population structure, namely no differentiation (panmictic), continuous change (isolation by distance) and distinct populations (distinct stocks) (see Figure 1.6). Based on the null hypothesis of a panmictic population (no genetic differentiation) distinct populations can be identified using an analysis of molecular variance (AMOVA) (Excoffier, Smouse, and Quattro 1992). This test compares the level of genetic variation among individuals of a population to the level of genetic variation between populations or sampling locations (Excoffier, Smouse, and Quattro 1992). The significance of any population differentiation is then established using permutational analyses (Excoffier, Smouse, and Quattro 1992).
A population structure with continuous change (isolation by distance) can be tested for by looking for a correlation between the pairwise level of genetic differentiation between individuals and the pairwise geographic distance (Meirmans, Meirmans, and Kirkendall 2012). A positive correlation will result when dispersal distances are smaller than a species’ range (Wright 1943).
A panmictic population (no genetic differentiation) can be tentatively concluded when no evidence is found to suggest a population is structured as distinct stocks or isolated by distance. However, this is not direct evidence of a panmictic population, instead a lack of evidence to reject the null hypothesis of no population structuring.
Another method for describing the population structure of a species is the construction of haplotype networks. Haplotype networks are widely used to visualise the relationships within or between populations or species (Paradis 2018). A number of methods for constructing haplotype networks exist, including parsimony, distances, maximum likelihood, Bayesian inference, split decomposition or consensus methods (see Holland et al. (2004); Paradis (2018)).
One distance based method of constructing a haplotype network is the minimum spanning haplotypes networks introduced by Bandelt, Forster, and Röhl (1999). This extends on the minimum spanning tree algorithm developed by Kruskal (1956) by allowing an integration of many minimum spanning trees rather than providing one minimum spanning tree. This method combines features of the algorithm by Kruskal (1956) for finding minimum spanning trees and the maximum-parsimony heuristic algorithm by Farris (1970; Bandelt, Forster, and Röhl 1999). Some methods of constructing haplotype networks will infer unobserved or ancestral haplotypes (Paradis 2018). However the minimum spanning network does not, providing the benefit of minimising the total length of the network when dealing with large datasets (Bandelt, Forster, and Röhl 1999).
Haplotype networks can have advantages over a phylogenetic analyses when it is suspected that there is an ancestral-decendance relationship among the haplotypes compared in the analysis, for example when using a DNA marker with a high mutation rate or when the samples may not all be contemporary (Paradis 2018).
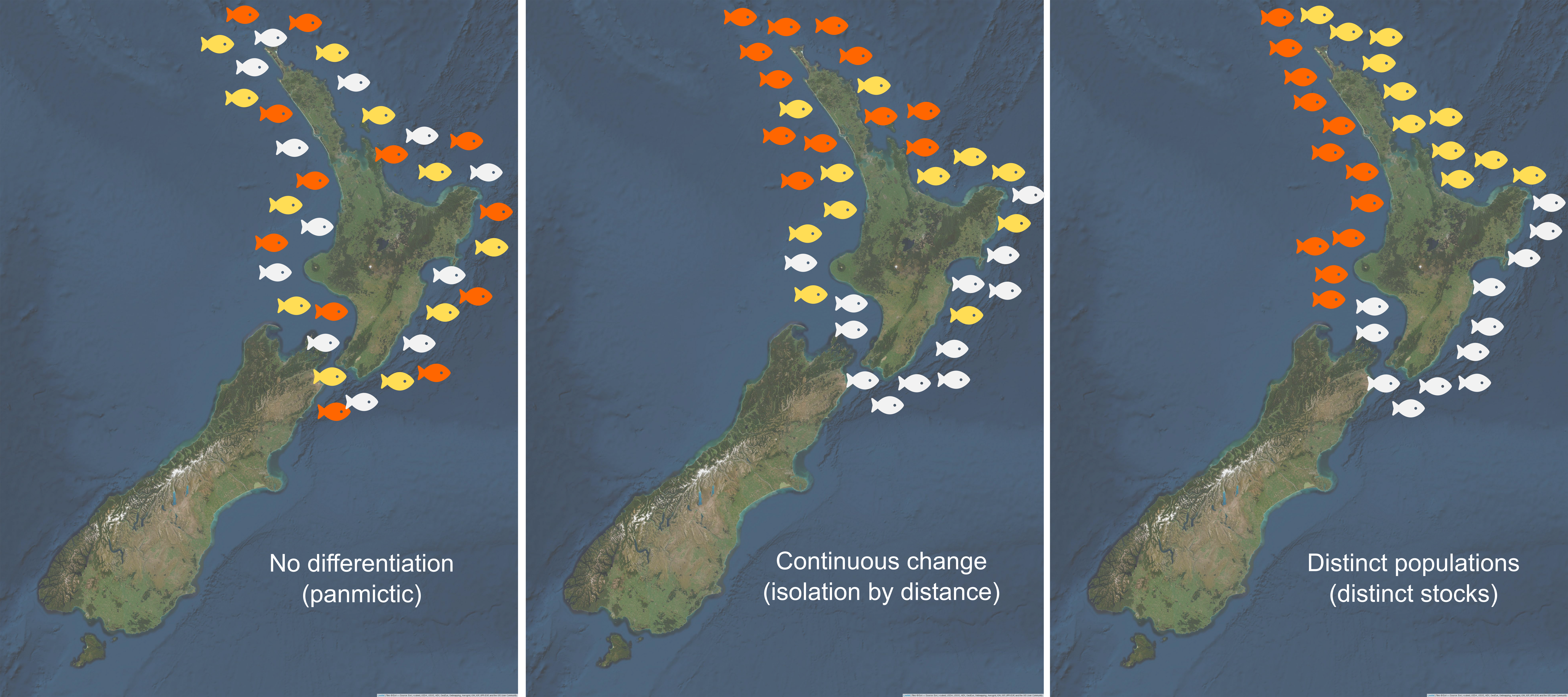
Figure 1.6: Three main types of genetic population structures (figure based on Laikre et al., 2005).
1.3.2.2 Demographic history
An excess of old mutations and/or a reduction of young mutations on a DNA region can result from several evolutionary forces, including natural selection, population bottlenecks or population expansions (Fu 1996). Therefore, the presence of evolutionary events in evolutionary lineage can be tested for by testing for departures from the neutral model (Fu 1996). Fittingly named, the neutral model assumes that a DNA marker is neutral and not undergoing selection (direct or indirect), recombination or that the population is undergoing a size expansion (Tajima 1989).
Based on the assumption that the number of sites on the DNA sequence is large and the DNA sequences are randomly sampled from a population, departures from the neutral model can be tested for with the Tajima D statistic (Tajima 1989). Both selection and a recent population bottleneck can produce a negative Tajima’s D value, although the types of genetic variation that will result from these evolutionary forces will be different. Natural selection will result in the maintenance of insertions and deletions, whereas a population bottleneck or population expansion will affect all types of DNA polymorphisms, resulting in the maintenance of nucleotide diversity (Tajima 1989) and therefore haplotype diversity.
The Fu’s F statistic is another statistical test developed more recently to test for departures from the neutral model (Fu 1996). It has the advantage of being more powerful than the Tajima’s D statistic at identifying selection or population expansions because it is more powerful for detecting departures from the neutral model (Fu 1996).
While Tajima’s D and Fu’s F statistics can indicate whether or not a populations have undergone a size expansion, mismatch distributions can estimate the size of populations over time. This is because changes in population size leaves characteristic signals in the distribution of nucleotide differences between pairs of individuals (Rogers and Harpending 1992; Rogers et al. 1996). Therefore comparing the observed distribution and expected distribution of pairwise nucleotide differences can indicate whether a population has experienced a bottleneck, undergone an size expansion (Rogers and Harpending 1992) or large spatial expansion (Ray, Currat, and Excoffier 2003).
The levels of migration between populations can be estimated from genetic data by estimating effective population sizes and immigration rates scaled by mutation (Beerli and Felsenstein 1999), described in this thesis as a ‘migration analysis’. This Bayesian approach integrates all possible relationships of P. georgianus based on the coalescent theory (Kingman 1982b, 1982a, 2000) and migration (Hudson 1990; Nath and Griffiths 1993; Nogueira et al. 1990).
1.4 Genetics and fisheries management
Understanding the population structure and taxonomy of fisheries species is an important basis on which fisheries are managed (Laikre, Palm, and Ryman 2005; Waples, Punt, and Cope 2008). This understanding allows implementation of fisheries management techniques that reduce the risk of genetic depletion by ensure fishing is undertaken on genetically homogeneous groups (Laikre, Palm, and Ryman 2005). Maintaining genetic diversity is crucial to the sustainability of fisheries (Laikre, Palm, and Ryman 2005). This is because genetic diversity is the basis of a species ability to adapt to environmental changes and complex interacting pressures (Allendorf and Ryman 2002). It can also reduce the risk of inbreeding depression which can lead to a reduction in productivity (Allendorf and Ryman 2002). Biologically informed fisheries management areas (ie. stocks) reduces the chance of over-exploitation of local populations which can result in local extinction or local reductions in genetic diversity (Laikre, Palm, and Ryman 2005).
Genetic tools are a comparatively cheap way to understand a species population structure, taxonomic status and genetic diversity, and in turn define fisheries management stocks (Bernatchez et al. 2017). It provides base-level measures that enable the sustainable utilisation of fishery resources (Bernatchez et al. 2017).
Like other New Zealand fisheries, the P. georgianus fishery is managed based on total allowable catch of P. georgianus stocks. Therefore, the first step in the successful management of this fishery is to understand what Pseudocaranx species are occurring in New Zealand’s P. georgianus fisheries. The next step is to understand the stock structure of New Zealand P. georgianus. Stock structure findings could have important implications for fisheries management by informing the inputs of the stock assessment model used as a basis for managing this fishery. Understanding the taxonomy and population stucture of P. georgianus will indicate whether a single or multiple population model is appropriate for New Zealand P. georgianus fisheries.
These questions can be addressed with genetic data. Phylogenetic analysis of a DNA marker with a species-level rate of variation can indicate which Pseudocaranx species are occurring in New Zealand. Population genetic analyses of a highly variable DNA marker provides fine-scale information on the population structure of P. georgianus. Exploring the demographic history of genetically distinct populations identified through population genetic analyses will provide further biological information regarding the population’s history and it’s response to historical events. In turn, this can provide insights into how P. georgianus populations will respond to future environmental pressures.
Although some genetic data has been produced for P. georgianus sampled from Australian waters, no genetic data is currently available in either GenBank or BOLD systems for New Zealand specimens of P. georgianus. Only one population genetic study has been carried out on a Pseudocaranx species (see Bearham (2004)) and no such studies have been undertaken on P. georgianus in New Zealand.
1.5 Research direction
This thesis will investigate the taxonomy, population structure, population connectivity and the demographic history of P. georgianus in New Zealand based on two mitochondrial DNA markers, the COI gene and the control region. P. georgianus from Australia will act primarily as a reference population for investigations of New Zealand P. georgianus. Other Pseudocaranx species will also be investigated to understand the taxonomic standing of P. georgianus. First, the mitogenome of P. georgianus will be assembled, primarily to act as a practical basis for producing genetic data by allowing primer design and identification of variable and conserved regions in the mitogenome. This research fits within a wider effort that seeks to broaden the application of genomic tools to inform the management and understanding of aquatic organisms (Bernatchez et al. 2017). The specific research questions are:
- What is the general structure and composition of the P. georgianus mitogenome and how does it compare to other fish species?
- What is the taxonomic status of P. georgianus in New Zealand?
- What is the population structure of P. georgianus in New Zealand?
- What is the level of population connectivity of P. georgianus within Australasia?
- What is the demographic history of New Zealand P. georgianus?
- What are the implications of these findings for the management of New Zealand P. georgianus fisheries?
- How do these findings compare to the findings of other New Zealand fisheries species?
References
Afonso, P., J. Fontes, K. Holland, and R. Santos. 2009. “Multi-Scale Patterns of Habitat Use in a Highly Mobile Reef Fish, the White Trevally Pseudocaranx Dentex, and Their Implications for Marine Reserve Design.” Journal Article. Marine Ecology Progress Series 381: 273–86. https://doi.org/10.3354/meps07946.
Afonso, P., J. Fontes, T. Morato, K. Holland, and R. Santos. 2008. “Reproduction and Spawning Habitat of White Trevally, Pseudocaranx Dentex, in the Azores, Central North Atlantic.” Journal Article. Scientia Marina 72 (2): 373–81.
Allendorf, F., and N. Ryman. 2002. “The Role of Genetics in Population Viability Analysis.” Book Section. In Population Viability Analysis, edited by S. Beissinger and D. McCullough, 50–85. Chicago: University of Chicago Press. https://doi.org/10.1016/bs.adgen.2017.09.007.
Australian Fisheries Management Authority. 2010. “Norfolk Island Inshore Fishery Data Summary 2006-2009.” Report. Australian Fisheries Management Authority.
Australian Government Department of Fisheries. 2019a. “Commonwealth Trawl and Scalefish Hook Sectors.” Web Page.
Australian Government Department of Fisheries. 2019b. “Norfolk Island Fishery.” Web Page.
Avise, J., J. Arnold, R. Ball, E. Bermingham, T. Lamb, J. Neigel, C. Reeb, and N. Saunders. 1987. “Intraspecific Phylogeography - the Mitochondrial Dna Bridge Between Population Genetics and Systematics.” Journal Article. Annual Review of Ecology and Systematics 18: 489–522. https://doi.org/10.1146/annurev.ecolsys.18.1.489.
Ballard, J., and M. Whitlock. 2004. “The Incomplete Natural History of Mitochondria.” Journal Article. Molecular Ecology 13 (4): 729–44. https://doi.org/10.1046/j.1365-294X.2003.02063.x.
Bandelt, H., P. Forster, and A. Röhl. 1999. “Median-Joining Networks for Inferring Intraspecific Phylogenies.” Journal Article. Molecular Biology and Evolution 16 (1): 37–48. https://doi.org/10.1093/oxfordjournals.molbev.a026036.
Bearez, P., and C. Villaroel. 2018. “First Record of Pseudocaranx Chilensis (Carangidae) from the Continental Coast of North-Central Chile.” Journal Article. Cybium 42 (3): 303–5. https://doi.org/10.26028/cybium/2018-423-009.
Bearham, D. 2004. “A mtDNA Study of the Population Structure of Silver Trevally Pseudocaranx Dentex and the Relationship Between Silver and Sand Trevally Pseudocaranx Wrighti in Western Australian Waters.” Thesis, Murdoch University.
Beerli, P., and J. Felsenstein. 1999. “Maximum-Likelihood Estimation of Migration Rates and Effective Population Numbers in Two Populations Using a Coalescent Approach.” Journal Article. Genetics 152 (2): 763–73. https://doi.org/10.1073/pnas.081068098.
Bernatchez, L., M. Wellenreuther, C. Araneda, D. Ashton, J. Barth, T. Beacham, G. Maes, et al. 2017. “Harnessing the Power of Genomics to Secure the Future of Seafood.” Journal Article. Trends in Ecology and Evolution 32 (9): 665–80. https://doi.org/10.1016/j.tree.2017.06.010.
Brooks, A. 2019. “Using Ecological Niche Modelling to Predict Climate Change Responses of Ten Key Fishery Species in Aotearoa New Zealand.” Thesis, Victoria University of Wellington.
Campos, P., M. de Castro, and A. Bonecker. 2010. “Occurrence and Distribution of Carangidae Larvae (Teleostei, Perciformes) from the Southwest Atlantic Ocean, Brazil (12-23 Degrees S).” Journal Article. Journal of Applied Ichthyology 26 (6): 920–24. https://doi.org/10.1111/j.1439-0426.2010.01511.x.
Casillas, S., and A. Barbadilla. 2017. “Molecular Population Genetics.” Journal Article. Genetics 205 (3): 1003–35. https://doi.org/10.1534/genetics.116.196493.
Castro, J., J. Santiago, and V. Hernandez-Garcia. 1999. “Fish Associated with Fish Aggregation Devices Off the Canary Islands (Central-East Atlantic).” Journal Article. Scientia Marina 63 (3-4): 191–98. https://doi.org/10.3989/scimar.1999.63n3-4191.
Cryer, M., P. Mace, and K. Sullivan. 2016. “New Zealand’s Ecosystem Approach to Fisheries Management.” Journal Article. Fisheries Oceanography 25: 57–70. https://doi.org/10.1111/fog.12088.
Department of Agriculture and Water Resources. 2018. “Fishery Status Reports 2018.” Report. Australian Government.
Dudley, B., N. Tolimieri, and J. Montgomery. 2000. “Swimming Ability of the Larvae of Some Reef Fishes from New Zealand Waters.” Journal Article. Marine and Freshwater Research 51 (8): 783–87. https://doi.org/10.1071/mf00062.
Dyer, B., and M. Westneat. 2010. “Taxonomy and Biogeography of the Coastal Fishes of Juan Fernandez Archipelago and Desventuradas Islands, Chile.” Journal Article. Revista de Biologia Marina Y Oceanografia 45: 589–617. https://doi.org/10.4067/s0718-19572010000400007.
Excoffier, L., P. Smouse, and J. Quattro. 1992. “Anaysis of Molecular Variance Inferred from Metric Distances Among DNA Haplotypes - Application to Human Mitochondrial DNA Restriction Data.” Journal Article. Genetics 131 (2): 479–91.
Fairclough, D., I. Potter, E. Lek, A. Bivoltsis, and R. Babcock. 2011. “The Fish Communities and Main Fish Populations of the Jurien Bay Marine Park.” Report. Centre for Fish; Fisheries Research.
Farmer, B., D. French, I. Potter, S. Hesp, and N. Hall. 2005. “Determination of Biological Parameters for Managing the Fisheries for Mulloway and Silver Trevally in Western Australia.” Report. Murdoch University.
Farris, J. 1970. “Methods for Computing Wagner Trees.” Journal Article. Systematic Zoology 19 (1): 83–92. https://doi.org/10.2307/2412028.
Fisheries Science Group. 2018. “Fisheries Assessment Plenary, May 2018: Stock Assessments and Stock Status.” Report. Fisheries New Zealand.
Fowler, A., R. Chick, and J. Stewart. 2018. “Patterns and Drivers of Movement for a Coastal Benthopelagic Fish, Pseudocaranx Georgianus, on Australia’s Southeast Coast.” Journal Article. Scientific Reports 8 (1): 16738–8. https://doi.org/10.1038/s41598-018-34922-6.
Francis, M., M. Morrison, J. Leathwick, C. Walsh, and C. Middleton. 2005. “Predictive Models of Small Fish Presence and Abundance in Northern New Zealand Harbours.” Journal Article. Estuarine Coastal and Shelf Science 64 (2-3): 419–35. https://doi.org/10.1016/j.ecss.2005.03.007.
Froese, R., and D. Pauly. 2019. “FishBase.”
Fu, Y. 1996. “New Statistical Tests of Neutrality for DNA Samples from a Population.” Journal Article. Genetics 143 (1): 557–70.
Holland, B., K. Huber, V. Moulton, and P. Lockhart. 2004. “Using Consensus Networks to Visualize Contradictory Evidence for Species Phylogeny.” Journal Article. Molecular Biology and Evolution 21 (7). https://doi.org/10.1093/molbev/msh145.
Horn, P. 1986. “Distribution and Growth of Snapper Chrysophrys Auratus in the North Taranaki Bight, and Managment Implications of These Data.” Journal Article. New Zealand Journal of Marine and Freshwater Research 20 (3): 419–30. https://doi.org/10.1080/00288330.1986.9516161.
Hudson, R. 1990. “Gene Genealogies and the Coalescent Process.” Journal Article. Oxford Surveys in Evolutionary Biology, 1–44.
Huelsenbeck, J., and B. Rannala. 2004. “Frequentist Properties of Bayesian Posterior Probabilities of Phylogenetic Trees Under Simple and Complex Substitution Models.” Journal Article. Systematic Biology 53: 904–13. https://doi.org/10.1080/10635150490522629.
Jaafar, T., M. Taylor, S. Nor, M. de Bruyn, and G. Carvalho. 2012. “DNA Barcoding Reveals Cryptic Diversity Within Commercially Exploited Indo-Malay Carangidae (Teleosteii: Perciformes).” Journal Article. Plos One 7 (11). https://doi.org/10.1371/journal.pone.0049623.
James, G. 1976. “Eggs and Larvae of the Trevally Caranx Georgianus (Teleostei: Carangidae).” Journal Article. New Zealand Journal of Marine and Freshwater Research 10 (2): 301–10. https://doi.org/10.1080/00288330.1976.9515614.
James, G. 1978. “Trevally and Koheru - Biology and Fisheries.” Journal Article. Fisheries Research Division Occasional Publication (New Zealand) 15: 50–54.
James, G. 1980. “Tagging Experiments on Trawl-Caught Trevally, Caranx Georgianus, Off North-East New Zealand, 1973-79.” Journal Article. New Zealand Journal of Marine and Freshwater Research 14 (3): 249–54. https://doi.org/10.1080/00288330.1980.9515867.
James, G., and A. Stephenson. 1974. “Caranx Georgianus Cuvier, 1833 (Pisces: Carangidae) in Temperate Australasian Waters.” Journal Article. Journal of the Royal Society of New Zealand 4 (4): 401–10. https://doi.org/10.1080/03036758.1974.10419384.
Kingman, J. 1982a. “On the Genealogy of Large Populations.” Journal Article. Journal of Applied Probability 19: 22–43.
Kingman, J. 1982b. “The Coalescent.” Journal Article. Stochastic Processes and Their Applications 14: 235–48.
Kingman, J. 2000. “Origins of the Coalescent: 1974-1982.” Journal Article. Genetics 156 (4): 1461–3.
Kress, W., C. Garcia-Robledo, M. Uriarte, and D. Erickson. 2015. “DNA Barcodes for Ecology, Evolution, and Conservation.” Journal Article. Trends in Ecology and Evolution 30 (1): 25–35. https://doi.org/10.1016/j.tree.2014.10.008.
Kruskal, J. 1956. “On the Shortest Spanning Subtree of a Graph and the Traveling Salesman Problem.” Journal Article. Proceedings of the American Mathematical Society 7: 48–50. https://doi.org/10.2307/2033241.
Laikre, L., S. Palm, and N. Ryman. 2005. “Genetic Population Structure of Fishes: Implications for Coastal Zone Management.” Journal Article. Ambio 34 (2): 111–19. https://doi.org/10.1639/0044-7447(2005)034[0111:Gpsofi]2.0.Co;2.
Langley, A. 2004. “Length and Age Composition of Trevally (Pseudocaranx Dentex) in Commercial Landings from TRE1 Purse-Seine Fishery, 2002-03.” Journal Article. New Zealand Fisheries Assessment Research Document Series.
Masuda, R., T. Kamaishi, T. Kobayashi, K. Tsukamoto, and K. Numachi. 1995. “Mitochondrial DNA Differentiation Between Two Sympatric Morphs of Striped Jack Near Japan.” Journal Article. Journal of Fish Biology 46 (6): 1003–10. https://doi.org/10.1111/j.1095-8649.1995.tb01405.x.
Meirmans, S., P. Meirmans, and L. Kirkendall. 2012. “The Costs of Sex: Facing Real-World Complexities.” Journal Article. Quarterly Review of Biology 87 (1): 19–40. https://doi.org/10.1086/663945.
Nath, H., and R. Griffiths. 1993. “The Coalescent in 2 Colonies with Symmetrical Migration.” Journal Article. Journal of Mathematical Biology 31 (8): 841–51. https://doi.org/10.1007/bf00168049.
National Institute of Water and Atmospheric Research. 2019. “Feature Layers by MPI Geospatial Management.” Web Page.
Neira, F., R. Perry, C. Burridge, J. Lyle, and J. Keane. 2015. “Molecular Discrimination of Shelf-Spawned Eggs of Two Co-Occurring Trachurus Spp. (Carangidae) in Southeastern Australia: A Key Step to Future Egg-Based Biomass Estimates.” Journal Article. Ices Journal of Marine Science 72 (2): 614–24. https://doi.org/10.1093/icesjms/fsu151.
Nogueira, N., M. Ferreira, N. Cordeiro, and P. Canada. 1990. “The Coalescent and the Genealogical Proccess in Geographically Structured Population.” Journal Article. Journal of Mathematical Biology 29 (1): 59–75. https://doi.org/10.1007/bf00173909.
Paradis, E. 2018. “Analysis of Haplotype Networks: The Randomized Minimum Spanning Tree Method.” Journal Article. Methods in Ecology and Evolution 9 (5): 1308–17. https://doi.org/10.1111/2041-210x.12969.
Ray, N., M. Currat, and L. Excoffier. 2003. “Intra-Deme Molecular Diversity in Spatially Expanding Populations.” Journal Article. Molecular Biology and Evolution 20 (1): 76–86. https://doi.org/10.1093/molbev/msg009.
Rogers, A., A. Fraley, M. Bamshad, S. Watkins, and L. Jorde. 1996. “Mitochondrial Mismatch Analysis Is Insensitive to the Mutational Process.” Journal Article. Molecular Biology and Evolution 13 (7): 895–902. https://doi.org/10.1093/molbev/13.7.895.
Rogers, A., and H. Harpending. 1992. “Population Growth Makes Waves in the Distribution of Pairwise Genetic Differences.” Journal Article. Molecular Biology and Evolution 9 (3): 552–69. https://doi.org/10.1093/oxfordjournals.molbev.a040727.
Rosenberg, N., and M. Nordborg. 2002. “Genealogical Trees, Coalescent Theory and the Analysis of Genetic Polymorphisms.” Journal Article. Nature Reviews Genetics 3 (5): 380–90. https://doi.org/10.1038/nrg795.
Smith-Vaniz, W., and H. Jelks. 2006. “Australian Trevallies of the Genus Pseudocaranx (Teleostei: Carangidae), with Description of a New Species from Western Australia.” Journal Article. Memoirs of Museum Victoria 63 (1): 97–106. https://doi.org/10.24199/j.mmv.2006.63.12.
Tajima, F. 1989. “Statistical Method for Testing the Neutral Mutation Hypothesis by DNA Polymorphism.” Journal Article. Genetics 123 (3): 585–95.
Waples, R., A. Punt, and J. Cope. 2008. “Integrating Genetic Data into Management of Marine Resources: How Can We Do It Better?” Journal Article. Fish and Fisheries 9 (4): 423–49. https://doi.org/10.1111/j.1467-2979.2008.00303.x.
Wright, S. 1943. “Isolation by Distance.” Journal Article. Genetics 28 (2): 114–38.
Yang, Z., and B. Rannala. 2012. “Molecular Phylogenetics: Principles and Practice.” Journal Article. Nature Reviews Genetics 13 (5): 303–14. https://doi.org/10.1038/nrg3186.